- ▪
Neuron: The primary cell of the PNS, which is polarized and has dendrites to receive information and axons to transmit information
- ▪
Nerves: Bundles of axons enclosed in specialized connective sheaths
- ▪
Schwann cell: Glial cell that supports and myelinates PNS axons
- ▪
Endoneurium: Collection of collagenous tissue that surrounds each individual axons and their associated Schwann cell
- ▪
Perineurium: The blood nerve barrier, which is composed of concentric layers of fibroblasts that form a sheath around each fascicle
- ▪
Epineurium: The collagenous connective tissue layer that surrounds the outer limits of the peripheral nerve, protecting it from external stress
- ▪
Monofascicular: Nerve section composed of one large fascicle
- ▪
Oligofascicular: Nerve section composed of a few fascicles
- ▪
Polyfascicular: Nerve section composed of many fascicles of varying sizes
- ▪
Neurapraxia: Nerve injury characterized by a reduction or complete block of conduction across a nerve lesion
- ▪
Axonotmesis: Nerve injury characterized by conduction block and interruption of axonal continuity
- ▪
Neurotmesis: Nerve injury characterized by conduction block, interruption of axonal continuity, and connective tissue damage
The human nervous system arguably defines the very nature of humanity. Through this complex and intricate system, effective communication and response is possible within the body and to the environment. This system has evolved to allow complex, coordinated movements while maintaining basic reflexes. These capabilities are facilitated by a variety of cells that make up the central nervous system (CNS)—brain and spinal cord and the peripheral nervous system (PNS). The PNS, the focus of this chapter, provides the mechanism for relaying information between the CNS and the environment.
One of the central paradigms of neuroscience has long been that the PNS, in contrast to the CNS, possesses the capacity of self-regeneration after traumatic injury. Despite advances in peripheral nerve repair techniques, however, complete functional recovery is seldom achieved in adults. In order for clinicians to better understand some of the limitations associated with the current treatment protocols for neural injuries, they need to be aware of the basic science behind peripheral nerve anatomy, injury, and healing. These topics are the focus of this chapter.
Relevant Developmental and Functional Anatomy
The origin of the PNS is from the ectodermal layer of the blastocyst, with most of the PNS cells being derived from the neural crest. The primary cell of the PNS is the neuron—a polarized cell with dendrites to receive information and axons to send information. Nerves are bundles of axons enclosed in specialized connective sheaths. Within the nerve, there are several different satellite cells known as glial cells, including Schwann cells, macrophages, and fibroblasts. Peripheral nerves can thus be defined as heterogeneous composite structures composed of neurons, Schwann cells, fibroblasts, and macrophages.
In 1839, Theodor Schwann identified the cell that now bears his name. Schwann cells, derived from the neural crest, are glial cells that support and myelinate PNS axons.
A mature, myelinating Schwann cell is responsible for extending a process of its plasma membrane to wrap an axon in myelin. As such, myelin is a multilamellar, membranous sheath that surrounds the axons of CNS and PNS nerves. Myelin itself acts as an insulator around the axon, reducing the dissipation of the action potential into the surrounding medium as it propagates through the axon. The myelin sheath has discontinuities along the nerve fiber known as nodes of Ranvier, which create distinct morphologic and biochemical domains along PNS nerves ( Fig. 42-1 ). Nodes of Ranvier contain high concentrations of voltage-gated sodium channels. The arrival of an action potential at a node depolarizes the membrane, opening the sodium channels to induce a massive influx of sodium ions into the axon. This generates an electrical pulse that is propagated down the axon to the next node of Ranvier, a process termed saltatory conduction. Saltatory conduction allows not only for an increased speed of nerve impulse transmission, but also reduces the energy requirement.

Unmyelinated and myelinated nerves are bundled together in fascicles, with three main layers of tissue: the endoneurium, perineurium, and epineurium ( Fig. 42-2 ). The endoneurium is a collection of collagenous tissue that surrounds each individual axon and their associated Schwann cells. The perineurium is an extension of the blood–brain barrier and is known as the blood–nerve barrier. It is composed of concentric layers of fibroblasts that form a sheath around each fascicle. Prominent basement membranes and numerous tight junctions control the intraneural environment by restricting diffusion into the fascicles. The perineurium is the neural layer most resistant to longitudinal traction and thus provides the peripheral nerve’s elastic properties. The epineurium is the collagenous connective tissue layer that surrounds the outer limits of the peripheral nerve protecting it from external stress. The epineurium is divided into two layers: the internal and external. The internal epineurium cushions the fascicles within the nerve, while the external epineurium protects the nerve from external environment.

Fascicular patterns are divided into the following three types: monofascicular, oligofascicular, and polyfascicular. Monofascicular patterns consist of one large fascicle, whereas oligofascicular patterns consist of a few fascicles; polyfascicular patterns consist of many fascicles of varying sizes that can be arranged with or without groupings of fascicles ( Fig. 42-3 ). Nerves found in the upper arm are routinely polyfascicular. In its course from the upper arm to the fingertips, a peripheral nerve undergoes changes from a polyfascicular pattern in the upper arm, oligofascicular in the elbow region, and monofascicular in the hand and fingers. For example, the ulnar nerve is polyfascicular as it exits the brachial plexus until just before the elbow, at which point it becomes oligofascicular. After the division into the motor branch at the wrist, the pattern is monofascicular. These patterns may help to determine which type of nerve repair is appropriate for a particular nerve injury. In surgical nerve repair, proper identification of fascicular arrangement is crucial to maximizing the chances for a successful outcome.

Peripheral nerves are extensively vascularized with separate yet interconnected microvascular systems in the epineurium, perineurium, and endoneurium. , The vascular pattern of the peripheral nerve is characterized by longitudinally oriented groups of vessels, with a great number of communicating anastomoses. The vasculature is composed of an intrinsic vascular system consisting of vascular plexa in the epineurium, perineurium, and endoneurium and an extrinsic system derived from closely associated vessels running with the nerve. The role of the intraneural microvascular system is vital in regard to the effects of chronic irritation, compression, mobilization, stretching, and transection, and should be considered in determining the proper surgical technique.
Classification of Nerve Injury
Nerve lesions are usually described using the standard classification schemes of Seddon or Sunderland ( Table 42-1 ). In 1943, Seddon distinguished between three grades of damage: neurapraxia, axonotmesis, and neurotmesis.
Injury | Pathophysiology | Exam Findings | Nerve Studies | Prognosis | Example |
---|---|---|---|---|---|
Neurapraxia (Seddon) | Reversible conduction block | Motor paralysis: complete but reversible | Distal nerve conduction: present | Good | Chronic nerve compression (CNC) injury |
1st Degree (Sunderland) | Localized segmental demyelination | Muscle atrophy: minimal | Motor unit action potential: absent | Full recovery usually within days to 2–3 weeks | e.g., Carpal tunnel syndrome |
Schwann cells dysfunction | Sensory alteration: minimal | Fibrillation: sometimes detectable | |||
Axonotmesis (Seddon) | Interruption of axon continuity | Motor paralysis: complete | Distal nerve conduction: absent | Fair | Crush injury |
2nd Degree (Sunderland) | Preserved nerve sheath | Muscle atrophy: progressive | Motor unit action potential: absent | Full recovery possible without surgery | |
Wallerian degeneration | Sensory alteration: complete | Fibrillation: present | Recovery at 1 mm/day | ||
3rd Degree (Sunderland) | Endoneurium disrupted | Same as 2nd degree | Same as 2nd degree | Same as 2nd degree | |
Epineurium and perineurium intact | |||||
4th Degree (Sunderland) | Endoneurium and perineurium disrupted | Same as 2nd degree | Same as 2nd degree | Same as 2nd degree | |
Epineurium intact | |||||
Neurotmesis (Seddon) | Axon, myelin, and connective tissue damaged | Same as 2nd degree | Same as 2nd degree | Poor | Transection injury |
5th Degree (Sunderland) | Disruption of endoneurium, perineurium, and epineurium | Surgery required | e.g., Lacerations | ||
Full functional recovery often not possible |
Neurapraxia is the simplest form of nerve injury and is characterized by a reduction or complete block of conduction across a nerve lesion, with preservation of conduction both proximal and distal to the lesion and conservation of axonal continuity. Neurapraxic conduction blocks are caused by localized segmental demyelination. Such demyelination is often mediated by progressive dysfunction of the Schwann cells, common in chronic nerve compression (CNC) disorders such as carpal tunnel syndrome. The prognosis for neurapraxic injuries is good.
Axonotmesis is a more severe form of nerve injury, which is characterized by interruption of axonal continuity with preserved integrity of the nerve sheath. Following injury, Wallerian degeneration occurs. Nerve crush injuries exemplify axonotmetic injuries.
Neurotmesis is the most severe form of nerve injury and occurs when the axon, myelin, and connective tissue components are damaged and disrupted or transected. In neurotmesis, recovery through regeneration cannot occur without surgical intervention. Such an injury can be created by incision, fracture, or laceration and is classified as neurotmesis.
In 1951, Sunderland devised a five-grade classification scheme, which is now more widespread than Seddon’s three-grade system. Roughly, grades I and II correspond to Seddon’s neurapraxia and axonotmesis, respectively. Sunderland further divided Seddon’s category of neurotmesis injuries into grades III, IV, and V, based on the extent of damage to the axonal supporting structures. In grade III injuries, axon continuity is disrupted by loss of endoneurial tubes, but the perineurium is preserved. In grade IV injuries, nerve fasciculi (axon, endoneurium, perineurium) are damaged and intraneural scarring occurs, but nerve sheath continuity is preserved. In grade V injuries, the endoneurium, perineurium, and epineurium are completely divided, and substantial perineural hemorrhage and scarring occurs.
Although not routinely used, additional modifications to the classification scheme have occurred more recently. In 1988, Mackinnon and Dellon presented a grade VI injury that represented a complex peripheral nerve injury, involving combinations of Sunderland’s grades of injury. Furthermore, in 1992, Millesi amended Sunderland classification to include the extent of associated fibrous tissue proliferation (type A fibrosis, type B fibrosis, type C fibrosis).
Traumatic/Acute Nerve Injury
Although the preceding classification scheme is helpful to the clinician, the basic science researcher classifies nerve injuries as simply acute or chronic. Acute and chronic nerve injuries lead to disparate physiologic and histopathologic changes to the involved nerve and its adjacent tissue, including demyelination, degeneration, remyelination, and regeneration.
Acute peripheral nerve injuries have a sudden onset, as in nerve crush and transection injuries. Almost immediately, the injured nerve undergoes a process known as Wallerian degeneration (WD), whereby the damaged segment of the nerve is phagocytosed, beginning at the first intact node of Ranvier and progressing distally to allow for regrowth to begin. Granular disintegration of the axonal cytoskeleton, the hallmark of WD, is triggered by increased production of axoplasmic calcium and proceeds in an anterograde fashion down the axon. By 48 to 96 hours after the injury, axonal continuity is lost and impulse conduction is blocked. By 36 to 48 hours afterward, myelin disintegration is well advanced. The clearance of axonal and myelin debris creates an environment hospitable for regeneration.
Schwann cells and macrophages play pivotal, intertwined roles in degeneration and regeneration after acute peripheral nerve injury. Soon after injury, activated Schwann cells secrete chemotactic factors to recruit hematogenous monocytes to the site of injury, where they differentiate into macrophages. The macrophage is the primary phagocyte of myelin and accumulates by 72 hours. Early on, the macrophages express major histocompatibility complex class II antigen 1a and are not phagocytic. , Later, the hematogenously derived macrophages penetrate the basal lamina, lose 1a expression, become phagocytic, and produce interleukin-1 (IL-1). , IL-1 stimulates Schwann cells to produce nerve growth factor (NGF), which is required for regeneration of axons and myelin formation.
Schwann cells play yet another role in regeneration. Whereas normal adult Schwann cells do not divide, Schwann cells within the distal segment divide within 24 hours of injury with peak response by 72 hours. They form Bünger’s bands, which are cytoplasmic processes that interdigitate and line up in rows under the original basal lamina of the nerve fiber. Regenerating axons travel within these channels to reach their target end-organ.
By 5 to 8 weeks after the injury, the degenerative process is usually complete; all that remains are nerve fiber remnants composed of Schwann cells within an endoneural sheath. Nonetheless, in more severe injuries, a more significant local reaction occurs, whereby hemorrhage and edema lead to a vigorous inflammatory response. Fibroblasts, glial cells that help maintain the extracellular matrix, proliferate, and a dense fibrous scar causes a fusiform swelling of the injured segment. In addition, interfascicular scar tissue develops, permanently enlarging the nerve trunk. While denervated, the endoneurial tubes begin to shrink, reaching a minimum size at 3 to 4 months after injury. , Concurrently, the endoneurial sheath progressively thickens secondary to collagen deposition along the outer surface of the Schwann cell basement membrane. , In severe injuries, numerous endoneurial tubes do not receive a regenerating axon, and progressive fibrosis ultimately obliterates them. ,
Changes in the neuronal cell bodies and nerve fibers proximal to the site of injury depend on the severity of the injury and the proximity of the injured segment to the cell body. In extreme cases, in which the cell body degenerates, the entire proximal segment undergoes WD and is phagocytosed. Arguably, the cell body and axon depend on each other for recovery: the cell body does not fully recover without the reestablishment of functional peripheral connections, and the final axonal caliber greatly depends on the recovery of the cell body. In response to axonal injury, the nucleus of the nerve cell body undergoes a process referred to as chromatolysis, whereby the nucleus migrates to the periphery of the cell and Nissl’s granules (nerve rough endoplasmic reticulum) break up and disperse. It is believed that chromatolysis somehow signals the perineuronal glial cells to proliferate and extend processes that interrupt synaptic connections and isolate the neuron for recovery. In addition, chromatolysis reprograms the metabolic machinery to enable the cell body to increase lipid and protein production necessary for regeneration. The reversal of chromatolysis is one of the earliest signs of regeneration.
In mild injuries the regenerative and repair processes begin almost immediately, but in more severe injuries nerve regeneration begins only after WD has run its course. One of the early signs of regeneration is the sprouting of new axons (growth cones) by myelinated and unmyelinated nerve fibers proximal to the injury site. Axons that successfully reinnervate the distal stump will mature. Magill and colleagues found that when the mouse sciatic nerve was crushed, 100% of motor end plates are denervated 1 week after crush, with partial reinnervation at 2 weeks, hyperinnervation at 3 and 4 weeks, and restoration of a 1 : 1 axon to motor end plate relationship 6 weeks after injury. Schwann cells help guide the regenerating axonal sprouts to the denervated motor end plates, reforming neuromuscular junctions. Depending on the size of the defect and extent of injury, axons may regenerate and begin to remyelinate at 6 to 8 weeks; however, the original thickness is never achieved. On average, axonal growth occurs at a rate of 1 to 2 mm/day with a decreased rate in distal regions. Nevertheless, in more serious injuries, prolonged denervation is observed, which can lead to muscle atrophy. Denervated muscle fibers atrophy quite rapidly (on average, 70% reduction of cross-sectional area by 2 months). Although not common, occasional dropout of muscle fibers does occur as a late phenomenon, between 6 and 12 months after denervation.
Healing of Acute Nerve Injury: Regeneration and Remyelination
Unlike CNS nerves, peripheral nerves are capable of spontaneous regeneration after injury. Regeneration occurs when the environment is permissive and the intrinsic growth capacity of neurons is activated. Functional regeneration requires both axon regrowth and remyelination by Schwann cells.
That functional recovery is often suboptimal for a number of reasons: (1) The correct organ does not innervate in the correct location. (2) The end organ may be degenerated by the time the axon reaches the target. (3) An incorrect receptor may be innervated. (4) The receptor may be in the wrong location. (5) Axon continuity may not be maintained.
Many of these issues are related to the surgical coaptation. Even under the ideal surgical conditions, results are suboptimal. As such, most surgeons recognize that molecular mechanisms for regeneration are not effective at producing normal functional regeneration without advances in medical intervention.
Regeneration
Activation of Intrinsic Growth Capacity
The activation of the intrinsic growth capacity is best studied in the dorsal root ganglion (DRG) primary sensory neurons. DRG neurons are pseudobipolar neurons that have only one axon stemming from the cell body, which branches out to two axons: the peripheral branch innervates the sensory organs in the peripheral tissues, whereas the central branch enters the spinal cord and ascends the dorsal column to terminate in the brain. Largely due to disparate environments, the peripheral branch spontaneously regenerates after injury, while the central branch does not. However, if the peripheral branch is injured before the central branch, a phenomenon known as a conditioning peripheral lesion occurs, whereby the central branch can regenerate beyond the injury site, in the inhibitory environment of the spinal cord. ,
The intrinsic growth capacity is controlled by a number of genes and gene products, proteins. Myelin-associated glycoprotein (MAG) is an important constituent of myelin in the PNS, where it is expressed primarily by promyelinating glial cells and plays a key role in the early stages on myelination. MAG has a biphasic function: early in development it enhances axonal growth, but in adults it inhibits axonal regeneration and neurite outgrowth. In adults, the binding of either myelin or MAG to the Nogo receptor (NgR)-p75 neurotrophic receptor complex activates the Rho pathway, which in turn inhibits cytoskeleton assembly.
In vitro experiments have shown that MAG inhibits DRG outgrowth. However, in vitro as well as in vivo experiments have shown that when the peripheral branch is lesioned, the DRG axons sufficiently activate the intrinsic growth capacity to overcome the inhibitory effects of MAG. , Two independent studies argue that disinhibition occurs because of the increased postlesional intracellular cyclic adenosine monophosphate (cAMP) levels. cAMP is synthesized from adenosine triphosphate and is a well-known second messenger, used for intracellular signal transduction. cAMP’s significance was discovered when injection of dibutyryl-cAMP (a cAMP analog) into a nonlesioned DRG resulted in regeneration of the central branch of DRG neurons.
The action of cAMP proceeds through protein kinase A (PKA). PKA refers to a family of enzymes whose activity is dependent on cAMP levels. Experiments have shown that if PKA is blocked, lesion-induced growth of neurons that have been plated on MAG is blocked. , The effects of both cAMP and PKA are transcription-dependent and are regulated by the cAMP response element binding (CREB) proteins. , CREB proteins are transcription factors that bind to certain sequences of DNA (Cre elements) to increase or decrease transcription of certain genes. When PKA triggers gene expression through CREB, the transcription of regeneration-related genes, such as Arginase I, is upregulated. Arginase I, the liver isoform of arginase, is an enzyme involved in polyamine synthesis (the urea cycle), and it has been discovered that cAMP disinhibition of MAG is ineffective when polyamine synthesis is blocked. It is hypothesized that polyamines may induce expression of other regenerative genes or may directly affect cytoskeleton organization. In sum, cAMP increases the transcription of Arginase I through PKA activation of CREB ( Fig. 42-4 ).
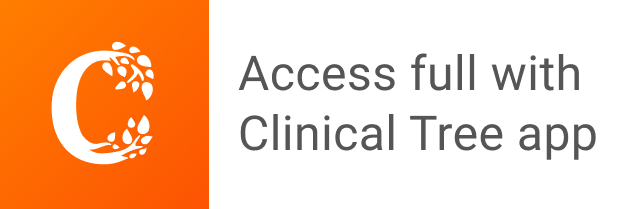