Features of Connective Tissue
- ▪
The outermost connective tissues of the nerve, the perineurium, and the epineurium provide diffusion barriers to keep toxins out of the nerve and offer biomechanical support to resist tension and compression of the nerve.
- ▪
The Schwann cell is a primary mediator of the neural response to prolonged compression resulting in morphologic changes to the cell and a loss of myelin.
Classification of Nerve Compression
- ▪
The accumulation of fluid (edema) within the endoneurial compartment increases endoneurial pressure and decreases blood flow to the nerve. This is the primary pathophysiology of nerve compression.
- ▪
Most nerve compression syndromes are type I (neuropraxia) injuries generating mild to moderate sensory symptoms. More severe injuries that include motor impairment are probably type II (axonotmesis).
- ▪
Nerve compression at one segment along a nerve pathway may increase vulnerability to nerve compression injury at a site distal or proximal to the segment. It is suspected that axoplasmic transport is disrupted along the pathway.
Critical to the diagnosis and treatment of nerve compression syndromes is a firm understanding of the anatomy, pathophysiology, and presentation of peripheral nerve lesions. Clinicians who treat patients with compressive neuropathies should specifically possess a strong understanding of the peripheral nervous system’s response to acute and chronic compression. In addition to clinical acumen, the health-care provider often relies on imaging and electrodiagnostic studies to assist in the diagnosis of peripheral nerve lesions. Once an accurate diagnosis has been established, the health-care team can devise a treatment plan that adequately addresses all potential sources of nerve compression.
Peripheral Nerve Pathway
As spinal nerve roots pass from the cervical spine through intervertebral foramina on their way to becoming peripheral nerves of the upper extremity, there are numerous sites of potential nerve compression. At each site, a potential mismatch exists between space available in the compartment and the contents of the compartment itself. Any decrease in the transectional area of the compartment or any volume increase in the contents of the compartment may result in restricted gliding between the tissues passing through that space. Any increase in intraneural pressure may then similarly impair both the extrinsic and intrinsic blood supply to the nerve tissue, ultimately resulting in a cascade of biochemical changes that result in functional deficit, pain, or anesthesia in the region that particular nerve supplies. Two of the most common and well-known sites of nerve compression are the carpal and cubital tunnels ( Fig. 47-1 ). Another common site of nerve compression occurs at the radial nerve with the “Saturday night palsy” because one may have a temporarily limited cognitive capacity to alleviate prolonged compression to the upper arm. The severity of clinical symptomatology that results from these classic compressive neuropathies is directly related to the degree of vascular insult, nerve swelling, and ultimately, the cumulative neurologic damage on a cellular level.

Peripheral Nerve Microanatomy
As is true in every facet of surgery and rehabilitation of the hand and upper extremity, a firm understanding of anatomy allows the health-care provider to truly appreciate the severity and implication of the pathologic process. The peripheral nervous system is arranged in such a way that a variety of tissue types function synergistically to relay motor and sensory stimuli to and from the central nervous system ( Fig. 47-2 ).

The main functional unit of the nervous system is the axon (nerve fiber) itself. Axons are themselves further bundled into fascicles, which in turn are organized into nerve trunks surrounded by a tough connective tissue matrix ( Fig. 47-3A ). Peripheral nerves possess three unique layers of connective tissue sheaths. From the outside inward, these layers are known as the epineurium, perineurium, and endoneurium ( Fig. 47-3B ). The epineurium is composed of fibroblasts and extracellular matrix consisting mostly of collagen as well as elastic fibers and mast cells. The epineurium provides structural support and biomechanical protection for nerve fascicles, acts as a diffusion barrier, and also contains blood vessels and adipose cells as supportive elements. Moving further inward, each fascicle is surrounded by perineurium, which is a mechanically strong membrane that resists tension and compression forces. It is more organized than the innermost layer, endoneurium, which itself is a sheath of connective tissue cells that surrounds a single axon. In addition to the structural integrity the perineurium provides to the nerve fascicle, it acts as a “blood–nerve barrier,” regulating and controlling the internal milieu of the nerve fascicles. Any breach or compression of the critical blood–nerve barrier results in the loss of normal homeostasis and ultimately leads to neural dysfunction. Leakage at the level of the endoneurium results in increased intraneural pressure and nerve fiber edema. Breakdown in the blood–nerve barrier results in the accumulation of protein and ingress of lymphocytes, fibroblasts, and macrophages, which ultimately initiates inflammation and eventually scar formation. Nerve fibers are thus surrounded and protected by a sophisticated network of supporting collagen structures that provide mechanical stability, nutrition, and homeostasis that allow the nerve to function normally. Any breach, internal derangement, or exogenous compression or tension will result in neural dysfunction.


Any discussion on the basic anatomy of the peripheral nerve system would be incomplete without reference to Schwann cells, the primary glial or supporting cells of the peripheral nervous system ( Fig. 47-4 ). These cells form the myelin sheath around axons by wrapping an extension of its plasma membrane around the nerve fiber. Continuing investigation into the processes contributing to and treating peripheral nerve damage has identified the myelin-forming Schwann cell as a primary mediator of the neural response to prolonged compression. A classic investigation by Ochoa et al. in the early 1970s provided the first description of the pathoanatomic changes in nerve myelin following nerve compression. Working with an animal model, the authors inflated a pneumatic cuff around the knee of adult female baboons creating a local conduction block in the medial popliteal nerve with subsequent leg and foot weakness. Electron microscope examination of sample nerve fibers from the region underneath the pneumatic cuff revealed a characteristic pattern of myelin disruption consisting of displacement of the node of Ranvier from its normal location. This displacement occurs with paranodal stretching of myelin on one margin of the node and invagination of the paranodal myelin on the other margin. The ruptured membrane essentially cleaves the paranodal myelin from its surrounding Schwann cell, creating an environment in which axonal conduction is disrupted and local demyelination occurs. The significance of Ochoa’s investigation was the identification of a nonischemic cause of diminished nerve conduction and localized axonal demyelination in the setting of neural compression.

Basic Nerve Physiology
Normal neural function depends on the propagation of electrical impulses termed action potentials from one cell to the next. A complicated, yet coordinated system of mechano-electrical events must occur for a nerve to function properly. Just as with any other cell, neural cells depend on adequate vascular inflow for optimal function.
The cell bodies of the somatic nervous system reside in the ventral horns (in motor nerves) or dorsal root ganglia (in sensory nerves), and function as the “workhorse” of the neural cell. Axons are the delicate peripheral extensions of the nerve cell body responsible for the propagation of an action potential. Together with surrounding helper cells or Schwann cells, the axons constitute nerve fibers, of which several subtypes exist. Group A nerve fibers denote the largest myelinated somatic afferent and efferent fibers, and have the fastest conduction velocity. Group B nerve fibers contain myelinated autonomic and preganglionic fibers, while group C consists of the thinnest, nonmyelinated visceral and somatic pain fibers, as well as pain fibers belonging to the sympathetic system.
Whatever the specific cell type, all nerves contain a biologic membrane that has a resting potential attributable to the separation of electric charges produced by the semipermeable nature of the membrane. This porous membrane excludes sodium but allows potassium to flow into the cell. An action potential is an “all-or-none” phenomenon in which an excitatory depolarizing event is initiated and a self-propagating signal is delivered from the cell body to the target end-organ. Current travels quickly down the length of the axon but is unable to flow across the myelinated segments of the nerve fiber, instead “jumping” from one internodal segment to the next. Myelinated nerve fibers therefore increase the velocity of propagation as the action potential quickly propagates down the axon jumping from internodal segment to the next, a process termed saltatory conduction .
Nutritional supply to nerve fibers is provided by a well-developed microvascular system that perfuses the epineurium, perineurium, and endoneurium. Peripheral nerves have a dual blood supply of intrinsic exchange vessels in the endoneurium and an extrinsic plexus of supply vessels in the epineurial space that crosses the perineurium to anastomose with the intrinsic circulation ( Fig. 47-5 ). A reduction or disruption of nerve blood flow may result in neural ischemia. Extrinsic vessels support smaller regional vessels that further subdivide to provide nutrition along the length of nerve fibers. When regional vessels approach the nerve trunk, they divide into ascending and descending branches running longitudinally in the epineurial layer. Inside the fascicles, a longitudinal pattern of capillaries run within the endoneurium. As the vessels pass through the innermost part of the perineurium into the endoneurium, they often cross in an oblique manner, making them quite vulnerable to external pressure.

Pathophysiology of Nerve Compression
Compared to complete peripheral nerve lesions, compressive neuropathies are unique as the nerve remains in continuity and there is no severance or tearing of the neural elements. The details of nerve degeneration and regeneration as a result of compression loading have been studied extensively over the past 30 years and have yielded a tremendous amount of information regarding the pathophysiology of nerve compression. In general, these studies demonstrate that nerve injury will relate to both the degree and duration of compression, with both mechanical and ischemic factors contributing to neurologic dysfunction.
Situations such as trauma or sustained compression will induce the accumulation of edema into the endoneurial space of the nerve trunk. Because of the diffusion barrier created by the perineurium and the lack of lymphatic vessels in the endoneurial space, the fluid may not easily escape. The result is an increase in endoneurial fluid pressure and encroachment of the normal endoneurial microcirculation. Lundborg et al. showed that following 2 to 8 hours of experimental compression-induced ischemia (80 mm Hg) in nerves of rabbits, the endoneurial fluid pressure may increase rapidly and persist for at least 24 hours. Another example of metabolic conduction block is the sensory loss and motor paralysis that can occur after deflating the tourniquet around the upper arm. This type of metabolic block, caused by local arrest of intraneural microcirculation, is immediately reversible when the compression is removed. With extended compression, however, edema within the fascicles can result in increased endoneurial pressure, which could compromise endoneurial capillary flow for hours or days, potentially permanently affecting function of the nerve.
Classification of Nerve Injuries
The success of the peripheral nerve repair process ultimately correlates with the extent and duration of compression. Clinically useful injury-grading systems have been developed that allow correlation of the microscopic changes occurring after nerve injury and patient symptomatology.
Neurapraxia, a type I injury, is the mildest form of injury and simply involves a conduction block. If there is anything to be seen histologically, it will be a segmental area of demyelination. The axon is not injured and therefore does not undergo regeneration. The majority of patients with compression neuropathies fall into this category. A type II injury is termed axonotmesis and will involve injury to the axon. Fibrillations would be seen with electromyography, along with a decrease in amplitude on electrodiagnostic testing consistent with loss of axons. This injury has the potential for eventual nerve regeneration. A type III injury is a more severe form of axonotmesis, as some degree of scar tissue forms in the endoneurium. Recovery is therefore less predictable. Patients with severe compression neuropathies fall into the category of type III damage. When the nerve is in continuity but completely blocked with scar tissue and no possibility of recovery, the injury pattern is suggestive of a type IV injury. A type V injury is the complete transection of the nerve. Types IV and V injuries would therefore not apply to compression neuropathy.
Interestingly, some patients with nerve compression such as carpal tunnel syndrome (CTS) have near-normal results on electrodiagnostic studies. Although diagnostic studies may in fact be negative, these patients will sometimes present with severe symptoms lending credibility to the theory of dynamic ischemia to the nerve rather than structural injury to the axon or its surrounding supporting cells.
Biologic Sequelae of Nerve Compression
Numerous methods have been used to induce acute or chronic compression of a peripheral nerve in animal models. For example, tourniquets have been applied around the limbs of rats, rabbits, and primates for varying amounts of time. In baboons, pressures as high as 500 to 1000 mm Hg have been achieved in an effort to induce structural nerve changes. Because of the difficulty in precisely controlling tourniquet compression, other researchers have applied a miniature inflatable cuff around a mobilized nerve to achieve more consistent compression for several hours. , These studies have shown that nerve injury is related to the degree and duration of compression, with mechanical and ischemic factors precipitating nerve dysfunction.
Acute Effects (Within Hours)
There have been several well-designed studies of healthy human volunteers in which extraneural pressure in the carpal tunnel was elevated for a maximum of 4 hours while nerve function was simultaneously monitored. , Pressure was precisely controlled with a clamp that applied an adjustable load to the palm, while the extraneural pressure in the carpal tunnel was measured with a fluid-filled catheter. Nerve function was blocked at 50 mm Hg, whereas some function was lost at 40 mm Hg. In subjects with different blood pressures, the critical extraneural pressure threshold above which nerve function was blocked was 30 mm Hg less than diastolic pressure. Combined with the observation that CTS may manifest with the treatment of hypertension, this finding provides another layer of support for an ischemic mechanism of acute nerve dysfunction.
Short-Term Effects (Within Days)
Human studies on the acute effects of nerve compression have demonstrated a rapid effect of intermediate pressure on nerve function; however, they have not addressed the effect of low extraneural pressure for an extended amount of time. Rydevik, Lundborg, and others have studied the effects of persistent intraneural edema in animals after short-term (order of days) compression at low pressures. Compression of 8 hours’ duration was shown to increase endoneurial pressure to a point that resulted in a reduction of intraneural blood flow. Interestingly, these studies also demonstrated that after low levels of compression for as little as 2 hours, endoneurial fluid pressures increased rapidly and persisted for at least an additional 24 hours after the compression was relieved. These long-lasting effects are probably due to the increased vascular permeability of the epineurial and endoneurial vessels after prolonged compression.
Long-Term Effects (Within Weeks)
To more closely mimic CTS, models of nerve compression have utilized silastic cuffs placed around the rat sciatic nerve and median nerve in primates. Not surprisingly, a dose–response relationship between the duration of compression and neural injury was observed. Initial changes showed a breakdown in the blood–nerve barrier, followed by subperineurial edema and fibrosis. Later changes included a localized, and later diffuse, demyelination, and finally Wallerian degeneration.
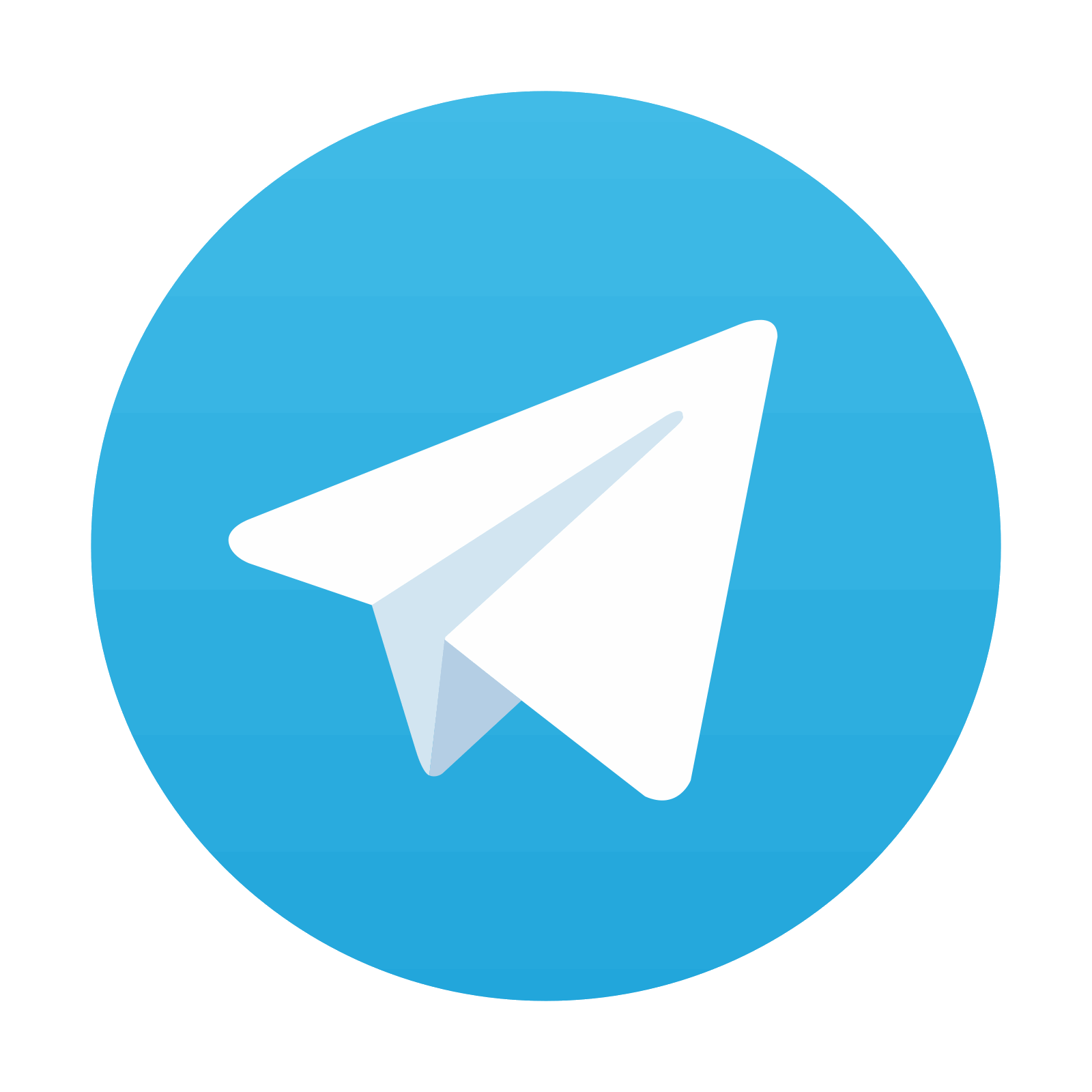
Stay updated, free articles. Join our Telegram channel
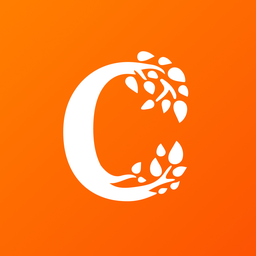
Full access? Get Clinical Tree
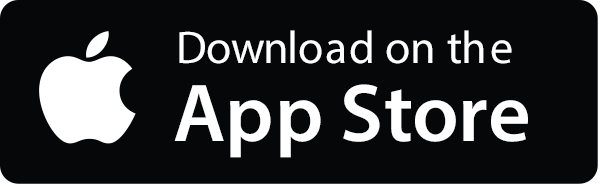
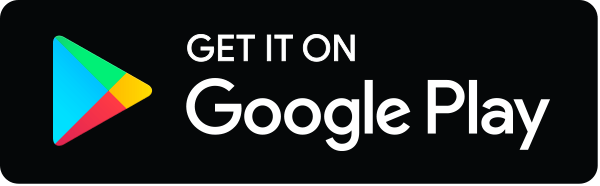