The central nervous system includes the brain and the spinal cord; the remainder belongs to the peripheral nervous system. The peripheral nervous system consists of two components: the somatic nervous system, which predominantly controls skeletal muscle (e.g., the “outer world”), and the autonomic nervous system, which predominantly controls smooth muscle, glands, and cardiac muscle (e.g., the “inner world”).1 The autonomic nervous system mainly includes sympathetic division (the so-called “fight-or-flight” response) and parasympathetic division (the “rest-and-digest” response).
This chapter provides a brief overview of the autonomic nervous system for the rehabilitation physician. This system plays a crucial role in adjusting the physiological state of the body to maintain circulatory homeostasis, or the stability of the “inner world.”2 The autonomic nervous system not only carries out the command of the brain, but also functions as a reflex circuit by using the sensory feedback of the organs to precisely adapt its output.3 The brain sets the balance of sympathetic and parasympathetic division based on the biological, physiological, or psychological situation, causing changes in the emphasis of the autonomic nervous system output (i.e., sympathetic dominant or parasympathetic dominant).3 If the balance is disturbed, either by behavior or by disease of the organ, this may lead to pathological changes that could affect the functioning of the whole individual.3 In general, excessive sympathetic activation is associated with specific medical conditions, such as hypertension, heart failure, myocardial infarction, sleep apnea, metabolic syndrome, kidney disease, polycystic ovary syndrome, gestational hypertension, and preeclampsia. In some situations, sympathetic inhibition and/or parasympathetic activation may be the underlying mechanisms, for example, neurally mediated syncope, postprandial hypotension, and multiple system atrophy (Fig. 7–1).
Figure 7–1
The autonomic nervous system. Schematic representation of the autonomic nerves and effector organs based on chemical mediation of nerve impulses. Blue-Grey (blue-grey line), cholinergic; light blue (light blue dotted), adrenergic; dark blue (dark blue dotted line) visceral afferent; solid lines, preganglionic; broken lines, postganglionic. The rectangle at right shows the finer details of the ramifications of adrenergic fibers at any one segment of the spinal cord, the path of the visceral afferent nerves, the cholinergic nature of somatic motor nerves to skeletal muscle, and the presumed cholinergic nature of the vasodilator fibers in the dorsal roots of the spinal nerves. The asterisk (*) indicates that it is not known whether these vasodilator fibers are motor or sensory or where their cell bodies are situated. (Reproduced with permission from Westfall TC, Westfall DP. Neurotransmission: The Autonomic and Somatic Motor Nervous Systems. In: Brunton LL, Chabner BA, Knollmann BC, eds. Goodman & Gilman’s: The Pharmacological Basis of Therapeutics, 12e New York, NY: McGraw-Hill; 2011.)

Endocrine glands are a primary effector of homeostatic control systems, which are regulated by the autonomic nervous system through efferent mechanisms that usually consist of both hormonal and neural elements.4 However, endocrine glands derived from neural tissue, such as the pineal and the adrenal medulla, are controlled by autonomic nerves to regulate secretion.4 Alterations in the function of the autonomic nervous system may influence hormones released by endocrine glands.
Figure 7–2 depicts the anatomy of sympathetic and parasympathetic division of the autonomic nervous system.
Cell bodies of the sympathetic division reside in the thoracic and lumbar portions of the spinal cord (T1–L2) and provide preganglionic efferent innervation to sympathetic neurons that are located in ganglia dispersed in three arrangements, including paravertebral, prevertebral, and previsceral or terminal ganglia.5 The preganglionic fibers are relatively short, and a single sympathetic preganglionic fiber may synapse with 20 or more postganglionic neurons. The postganglionic fibers are long and terminate in several visceral organs (effectors). The axons of the presynaptic neurons are myelinated, while the axons of the postsynaptic neurons are generally unmyelinated and of small diameter (e.g., <5 μm).5 The target organs of the sympathetic division include smooth muscle, cardiac muscle, glandular structures, parenchymal organs (e.g., liver, kidneys, bladder, reproductive organs), and other skin/cutaneous structures5 (Fig. 7–3).
Figure 7–3
The sympathetic nervous system. Organ innervation, receptor type, and response to stimulation. The origin of the sympathetic chain is the thoracoabdominal (T1–L3) spinal cord, in contrast to the craniosacral distribution of the parasympathetic nervous system. Another anatomic difference is the greater distance from the sympathetic ganglion to the visceral structures. (Reproduced with permission from Chapter 14. Adrenergic Agonists & Antagonists. In: Butterworth JF IV, Mackey DC, Wasnick JD, eds. Morgan & Mikhail’s Clinical Anesthesiology, 5e New York, NY: McGraw-Hill; 2013.)

Cell bodies of the parasympathetic division are located in the brain (cranial nerves III, VII, IX, and X) and in the sacral region of the spinal cord (sacral spinal nerves S2–S4). Preganglionic fibers are long, whereas postganglionic fibers are short (on or near the target viscera). Presynaptic neuron of the parasympathetic division may synapse four to five postsynaptic neurons, which supply a single visceral effector. The most caudal cranial nerve involved in the preganglionic parasympathetic division is the vagus nerve (cranial nerve X).5 The dorsal motor nucleus of the vagus is located in the medulla and sends preganglionic fibers to innervate all organ systems within the chest, abdomen, and pelvic regions. The glossopharyngeal (cranial nerve IX) and vagus nerves also contain a substantial number of afferent fibers, which provide a critical component of the baroreceptor reflex arc relaying information regarding the systemic blood pressure to central cardiovascular areas in the nucleus of the tractus solitaries (NTS) and other medullary centers involved in blood pressure and heart rate control5 (Fig. 7–4).
Figure 7–4
The parasympathetic nervous system uses acetylcholine as a preganglionic and postganglionic neurotransmitter. (Reproduced with permission from Chapter 12. Cholinesterase Inhibitors & Other Pharmacologic Antagonists to Neuromuscular Blocking Agents. In: Butterworth JF IV, Mackey DC, Wasnick JD, eds. Morgan & Mikhail’s Clinical Anesthesiology, 5e New York, NY: McGraw-Hill; 2013.)

The autonomic nervous system, through its modulation of the sympathetic-parasympathetic balance and neurohumoral secretion, plays a crucial role in cardiovascular and respiratory control, thermal regulation, gastrointestinal motility, urinary and bowel excretory functions, reproduction, and metabolic and endocrine physiology.5
The autonomic nervous system functions by releasing the neurotransmitters acetylcholine and norepinephrine and the hormone epinephrine.6 Acetylcholine is the neurotransmitter released at all preganglionic sites, both sympathetic and parasympathetic. Postganglionic sympathetic fibers release norepinephrine upon stimulation, but the postganglionic sympathetic innervation to the sweat (somatic) glands is cholinergic. All postganglionic parasympathetic fibers release acetylcholine when stimulated. Figure 7–5 depicts neurotransmitters and the hormone released by preganglionic and postganglionic axons of the sympathetic and parasympathetic divisions.
Acetylcholine binds to nicotinic receptors on the cell bodies of all preganglionic nerves and postganglionic parasympathetic nerves; however, it binds to muscarinic receptors in the target organs. Acetylcholine-mediated effects in the body include increasing the tone of the urinary bladder and bowel, increasing gastric acid secretion, stimulating salivation and tear production, increasing sweating, and decreasing the rate and force of the heartbeat.6 Norepinephrine binds to alpha- (both alpha-1 and alpha-2) and beta-adrenoceptors (mainly beta-1). Norepinephrine plays an important role in the regulation of blood pressure and heart rate during daily activities, such as postural change, walking, jogging, and weight lifting. Epinephrine binds to both alpha- and beta-adrenoceptors (mainly beta-2). It helps maintain organismic integrity during various challenges such as hypoglycemia, hypothermia, hemorrhage, hypotension, anoxia, and emotional distress6 (see Figs. 7–3 and 7–4).
Adenosine 5′-triphosphate, neuropeptide Y, and 5-hydroxytrytamine have been suggested to be co-transmitters with norepinephrine and/or acetylcholine in sympathetic and parasympathetic nerves, which implies more sophisticated local control mechanisms than were envisaged in earlier times.7
In humans and other species, nerves presumed to function as baroreceptors are most abundant in the adventitia of the carotid sinus and aorta.8 The corresponding afferent baroreceptor activity is transmitted to the NTS in the medullary brainstem via carotid sinus and glossopharyngeal nerves, and aortic depressor and vagus nerves, respectively9 (Fig. 7–6).
Figure 7–6
The baroreceptor reflexes : Components of the arterial baroreceptor reflex pathway. nts, nucleus tractus solitarius; rvlm, rostral ventrolateral medullary group; rn, raphé nucleus; na, nucleus ambiguus; ??, incompletely mapped integration pathways that may also involve structures outside the medulla. (Reproduced with permission from Regulation of Arterial Pressure. In: Mohrman DE, Heller L, eds. Cardiovascular Physiology, 9e New York, NY: McGraw-Hill; 2018.)

The afferent input signals are integrated and replayed through a network of the central nervous system neurons, which control efferent sympathetic and parasympathetic nerve activities to the heart, resulting in changes in heart rate and myocardial contractility, and efferent sympathetic nerve activity to the kidney and peripheral vasculatures, resulting in changes in vascular tone in the renal and skeletal muscle beds, as well as (de)activation of the renin-angiotensin-aldosterone system. With the microneurographic technique, postganglionic efferent sympathetic outflow to the skeletal muscle vasculature can be recorded as muscle sympathetic nerve activity (MSNA) (Fig. 7–7).10,11 MSNA plays an essential role in vasoconstriction in the skeletal muscle and contributes importantly to blood pressure regulation during daily activities in humans. The baroreceptor afferent inputs to the central nervous system also cause release of the vasoconstrictor and antidiuretic peptide vasopressin from the posterior pituitary gland.9 The arterial baroreflex is usually characterized as a negative-feedback system that attempts to lower blood pressure when it is high and raise blood pressure when low.12
Cardiopulmonary, or “low-pressure,” baroreceptors are a group of mechanoreceptors innervating the heart, vena cava, and pulmonary vasculature.9 These receptors are sensitive to changes in central blood volume. The afferent inputs, the central nervous system integration, and efferent pathways are similar to that of the arterial baroreflex (but not identical).9 Changes in central blood volume elicit changes in cardiopulmonary baroreceptor activity, leading to reflex changes in efferent sympathetic nervous activity (SNA), vascular resistance, and renin and antidiuretic peptide vasopressin release. It is suggested that cardiopulmonary baroreflex has little impact on heart rate.
The adrenal gland offers a crosstalk between the endocrine and autonomic nervous systems. The adrenal cortex is primarily regulated by the hypothalamic-pituitary-adrenocortical axis, whereas the adrenal medulla is primarily under sympathetic neural control.5 Both adrenal cortex and medulla respond to stress and metabolic aberrations with increases in plasma cortisol and catecholamines to ensure the needed neurohumoral adaptations4,5 (Fig. 7–8). Another example of the autonomic-endocrine interaction is the ovarian secretion of steroids, including estrogen and progesteron.4 In addition to hypothalamic-pituitary control, the ovary receives sympathetic and parasympathetic innervation to regulate ovarian function.13 For both the adrenal cortex and the ovary, the secretory response to their tropic anterior pituitary hormone is increased by SNA.4 Sympathetic nerves release norepinephrine and neuropeptides that act indirectly by changing organ blood flow to alter the exposure of the endocrine cell to pituitary hormones or directly by modulating endocrine cell steroidogenesis. Similar to the adrenal cortex and the ovary, the testes and the thyroid receive input from both the anterior pituitary and the antidiuretic peptide vasopressin.
Figure 7–8
Hypothalamic-pituitary-adrenal axis. Corticotropin-releasing factor (CRF), produced by the hypothalamus and released in the median eminence, stimulates the synthesis and processing of proopiomelanocortin, with resulting release of proopiomelanocortin peptides that include adrenocorticotropic hormone (ACTH) from the anterior pituitary. ACTH binds to the melanocortin-2 receptor in the adrenal gland and stimulates the cholesterol-derived synthesis of adrenal steroid hormones. Glucocorticoids released into the systemic circulation exert negative feedback inhibition of CRF and ACTH release from the hypothalamus and pituitary, respectively, in a classic example of negative feedback hormone regulation. This closely regulated circuit is referred to as the hypothalamic-pituitary-adrenal (HPA) axis. (Reproduced with permission from Chapter 6. Adrenal Gland. In: Molina PE, eds. Endocrine Physiology, 4e New York, NY: McGraw-Hill; 2013.)

Daily physiological activities (e.g., upright posture, walking, jogging, weight lifting) and psychological (mental) stress all pose challenges to circulatory homeostasis in humans.12 Mean arterial pressure, which is determined by stroke volume, heart rate, and total peripheral resistance, is a critical hemodynamic factor for circulatory homeostasis, and it is regulated acutely through the reflex mechanisms in order to maintain appropriate perfusion of vital organs.14
Human beings spend a large portion of the day in the upright posture (orthostasis). Gravity shifts 500 to 800 mL of blood from the thorax to the lower body, and thus, venous return is reduced and central blood volume is decreased, leading to reductions in stroke volume and cardiac output, a fall in arterial pressure, and ultimately occurrence of syncope if reflex circulatory adjustments are inappropriate.12,15 Both arterial and cardiopulmonary baroreceptors are unloaded simultaneously during upright posture, resulting in decreased afferent inputs to the central nervous system, parasympathetic withdrawal and sympathetic activation to the heart (an increase in heart rate), and sympathetic activation to peripheral vasculatures (an increase in total peripheral resistance). Therefore, mean arterial pressure is maintained through the baroreflex mechanisms in healthy individuals.
The orthostatic response is classified into three stages: the initial response (first 30 seconds), the early steady-state circulatory adjustment (after 1 to 2 minutes upright), and prolonged orthostasis (at least 5 minutes upright).16 It is believed that the initial and early steady-state circulatory adjustments are controlled predominantly by the autonomic nervous system, and sympathetic adrenergically mediated vasoconstriction, rather than an increase in heart rate, plays a crucial role in arterial pressure maintenance. During prolonged orthostasis, activation of the sympathetic division as well as the renin-angiotensin-aldosterone system is essential in the maintenance of mean arterial pressure.16
Rhythmic or dynamic exercise (e.g., walking, cycling, running) and static or isometric exercise (e.g., weight lifting) are two major forms of exercise, though many activities include components of both. The cardiovascular response during both rhythmic and static exercise is initiated by a feed-forward mechanism, termed “central command,” which involves higher brain centers such as the motor cortex, hypothalamic, and mesencephalic locomotor regions that activate parallel circuits controlling locomotor, cardiovascular, and ventilatory functions.17 Central command is responsible for the immediate increases in heart rate, blood pressure, and respiration seen at the onset of exercise.12 As exercise continues, both mechanical and metabolic signals from active skeletal muscle provide feedback to cardiovascular centers in the brain through group III and IV muscle afferents, the so-called “exercise pressor reflex,” to precisely match systemic oxygen delivery with metabolic demand.17,18
Contracting (exercising) skeletal muscle produces vasodilatory metabolites (i.e., adenosine, prostaglandins, nitric oxide), which attenuate sympathetically mediated vasoconstriction, and this phenomenon is known as “functional sympatholysis.”19–21 However, it has been found that nitric oxide is not obligatory for functional sympatholysis in contracting skeletal muscles of healthy humans.21 Enhanced endothelium-derived hyperpolarizing factor activity in conditions of nitric oxide deficiency may contribute to functional sympatholysis.22 Vascular resistance in active skeletal muscle decreases to facilitate increases in muscle perfusion, while in inactive skeletal muscle vascular resistance increases so that systemic arterial pressure can be supported.19,23 If sympathetically mediated vasoconstriction is impaired, for instance, in patients with autonomic failure, hypotension will occur during exercise, and at times, this hypotension may be profound. Additionally, cardiac output increases proportionate with oxygen uptake, thus allowing the maintenance or, in most cases, an increase in mean arterial pressure.
There is a parallel increase in arterial blood pressure and heart rate at the beginning and during exercise, indicating a resetting of the arterial baroreflex. Upward resetting of the operating point of the arterial baroreflex appears to be the major factor responsible for the sympatho-excitatory response and increasing blood pressure during exercise.24 It has been proposed that central command resets the arterial baroreflex and in turn raises cardiac output by sudden withdrawal of tonic outflow to the heart.25 Conversely, activation of the exercise pressor reflex may also contribute to the resetting of the arterial baroreflex26,27 (Fig. 7–9).
During psychological stress (e.g., depression), the sympathetic division is activated and parasympathetic division is inhibited, and therefore, both heart rate and blood pressure usually increase. When stress level increases, epinephrine is released from the adrenal medullae. As stress increases even further, corticotropin-releasing factor activates the sympathetic nervous system and leads to the release of adrenocorticotropic hormone and adrenocortical steroids.28 Prolonged or sustained psychological stress may lead to the development of cardiovascular disease through the autonomic nervous system (Fig. 7–10).
Figure 7–10
Pathophysiology of depression and cardiovascular disease. (Reproduced from Chapter 96. Effects of Mood and Anxiety Disorders on Cardiovascular Disease. In: Fuster V, Walsh RA, Harrington RA, eds. Hurst’s The Heart, 13e New York, NY: McGraw-Hill; 2011.)

Individuals may experience an increase (positive responders) or a decrease (negative responders) in MSNA during psychological stress. It has been found that negative MSNA responders exhibit a more rapid rise in diastolic pressure at the onset of the stressor, suggesting a baroreflex-mediated suppression of MSNA. However, in positive responders there is a more sluggish rise in blood pressure during psychological stress, which appears to be MSNA-driven.29 These results suggest that whether MSNA has a role in the pressor response is dependent on the reactivity of blood pressure early in the task.29
Normal aging affects autonomic circulatory control.30 For example, numerous studies have shown that heart rate variability and heart rate responses during various physiological stimulations are smaller in older people compared with younger people. These results suggest that parasympathetic control of heart rate is impaired with age. In contrast, there is an age-related increase in sympathetic activity (e.g., increases in plasma norepinephrine concentration, MSNA, and peripheral vascular resistance).31 Impaired baroreflex function caused by large-artery stiffening may explain, at least in part, the age-related change in the autonomic nervous system.32 Despite the increase in sympathetic activity, cardiac and vascular responsiveness may be blunted with age. Orthostatic and postprandial hypotension are two most common manifestations of age-related impairment in autonomic circulatory control.30,33–35 Both are defined as at least 20 mm Hg reduction in systolic blood pressure on moving from supine to upright or within 1 hour of eating a meal.30
There are sex-specific differences in autonomic circulatory control. On average, young women have lower arterial blood pressure and MSNA when compared with young men, which may be attributed to female sex hormones, particularly estrogen.31,36–39 Blood pressure and MSNA increase with age, and the increment is greater in women than in men.40–44 Therefore, older women (e.g., ≥65 years) have a similar or even greater basal MSNA and a greater increase in arterial pressure for a given increase in MSNA.31,32,40,41,45,46 The latter may be one important mechanism for the higher prevalence of hypertension in older women.47 Baroreflex control of MSNA (or sympathetic baroreflex sensitivity) has been reported to be similar between young men and young women.36,45,46,48 With women of more advanced age, sympathetic baroreflex sensitivity remains unchanged43,46 or decreases.45,49 It has been found that sympathetic baroreflex sensitivity is lower in older women than in older men, which appears to be associated with greater arterial stiffness in women,32 perhaps leading to less baroreceptor distortion for a given pressure pulse.
The medical history is crucial in the recognition and evaluation of dysfunction or impairment of the autonomic nervous system. It should include (1) chief compliant, (2) history of the present illness, (3) a complete listing of all medications and supplements, (4) past medical history, (5) family history, and (6) a full system review with particular reference to autonomic symptoms.50,51 Common symptoms of autonomic dysfunction or impairment may include, but are not limited to, palpitations, nausea, vomiting, vertigo, blurred vision, weakness, tremulousness, pallor, coldness, incontinence, constipation, erectile failure, etc.
In addition to medical history, the physical examination is essential to ascertain whether autonomic dysfunction or autonomic disorders are present and what systems are involved.50 The general physical examination may include blood pressure, heart rate, temperature, skin color, sweating, joint function, pupils, etc.
Some symptoms of autonomic dysfunction can easily be evaluated at the bedside in a clinical setting. With continuously monitoring of the heart rate by the electrocardiogram, heart rate variability with normal or deep breathing can be assessed. In addition, heart rate and blood pressure responses to active standing can be used for assessment of autonomic function.
The specific aims of clinical evaluation include the following50: (1) to recognize the presence and distraction of autonomic dysfunction; (2) to recognize patterns of autonomic failure that can be related to specific syndromes; (3) to recognize potential treatable disorders; (4) to recognize disorders that warrant further evaluation; (5) to probe the diverse areas involved with dysautonomia and their numerous manifestations that can defy laboratory assessment; (6) to evaluate autonomic dysfunction as a function of time; and (7) to evaluate the effect of autonomic dysfunction on the system and the patient.
Laboratory autonomic function tests may include, but are not limited to, deep breathing, carotid sinus massage, Valsalva maneuvers, the cold pressor test, rhythmic or static handgrip exercise, head-up tilt test or standing, and sweat tests. Measurements may contain blood pressure, heart rate, stroke volume, cardiac output, total peripheral resistance, respiration, plasma catecholamine concentration, MSNA with the microneurographic technique if available,11 skin temperature, or sweating patterns. The purpose and a brief description of each test are shown in Table 7–1.
Testing | Purpose | Procedures | Outcome Variables |
Controlled breathing and deep breathing | Assess HRV, BPV, arterial BRS | Patient breathes at a fixed rate (e.g., 12 breaths per minute or 0.2 Hz) for 5–10 min during controlled breathing and six breaths per minute (0.1 Hz) for 1 min during deep breathing |
|
Valsalva maneuver | Assess baroreflex control of HR, and sympathetic vasoconstriction | Patient blows through a mouthpiece against a resistance (30–40 mmHg) for 10–20 sec |
|
Carotid sinus massage | Assess arterial BRS | The investigator massages the carotid bulb of the patient for 5 sec on one side and then the other side |
|
The cold pressor test | Assess central vasomotor integration and efferent sympathetic pathways | The patient’s hand up to the wrist is immerged in a 4°C ice-water bath for 2 min, followed by 5–10 min of recovery |
|
Static handgrip | Assess the exercise pressor reflex (mechano- and metabo-reflex), central command, and baroreflex | The patient performs static handgrip at 30%–40% of MVC until fatigue, followed by 2 min of PECA with an upper arm cuff inflated to 200 or 250 mmHg |
|
Active standing | Assess autonomic circulatory control during orthostatic stress | Patient stands upright for a period of time (ranging from 5 min up to 2 hr) |
|
Head-up tilt | Patient is passively tilted upright to 60 or 70 degrees for a period of time (ranging from 5 to 45 min) | ||
Modified Oxford method | Assess cardiovagal and sympathetic baroreflex function | Briefly, an intravenous bolus infusion of sodium nitroprusside (100 μg), followed 60 sec later by phenylephrine hydrochloride (150 μg) |
|
QSART | Assess integrity of postganglionic sympathetic sudomotor axon (useful in monitoring a neuropathy) | Electrodes filled with ACh are placed on the leg and wrist; a mild electrical current (iontophoresis) is applied to help the drug stimulate the sweat glands, which evokes sweating at the site |
|
The aims of laboratory assessment are as follows52: (1) to detect the presence of autonomic failure; (2) to quantitate its severity and the apportion of the type (adrenergic, cardiovagal, or sudomotor) of deficits; (3) to determine the distribution of autonomic failure; (4) to determine the site of autonomic lesion; and (5) to detect the presence of altered sympathetic effect.
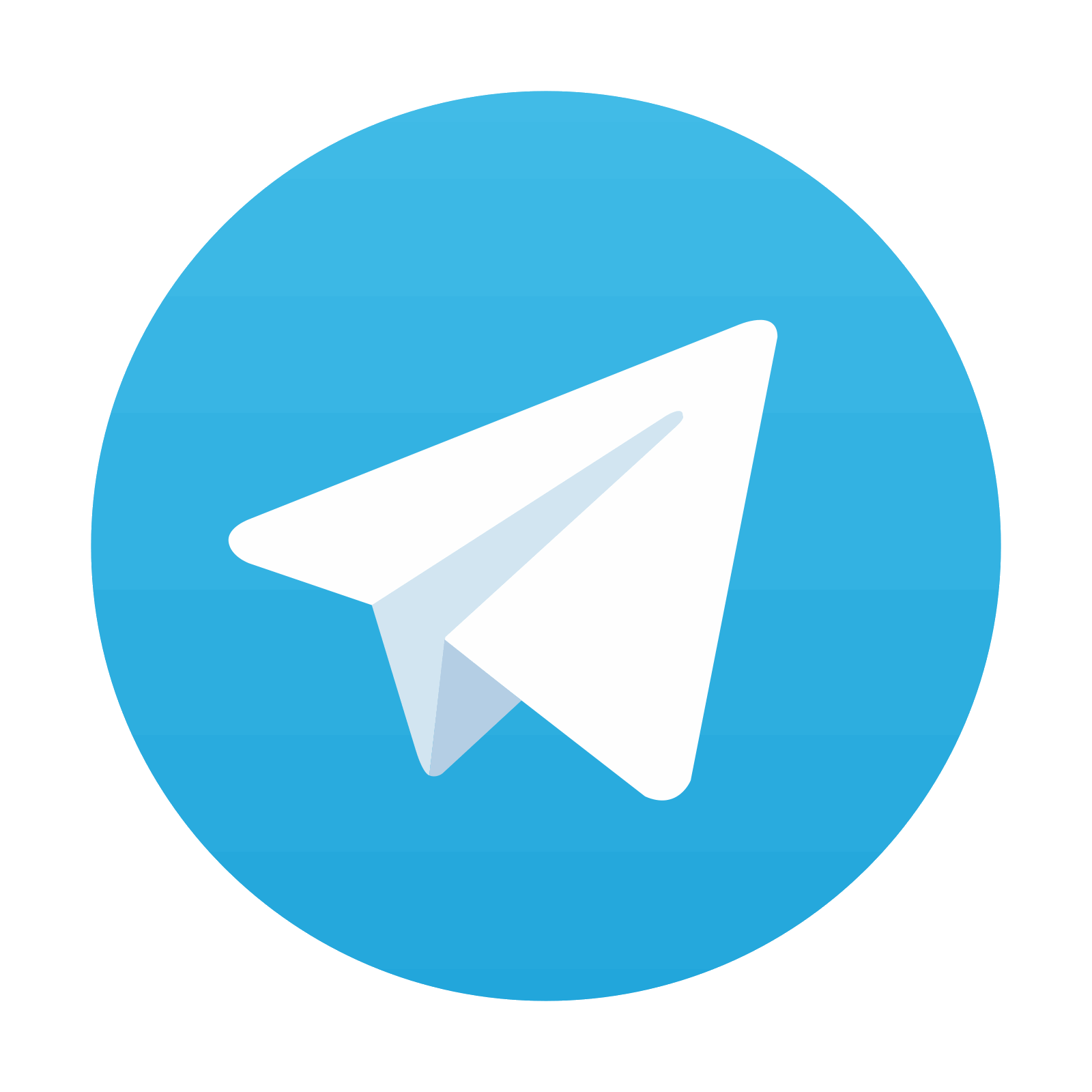
Stay updated, free articles. Join our Telegram channel
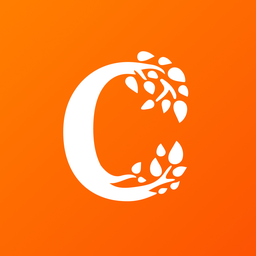
Full access? Get Clinical Tree
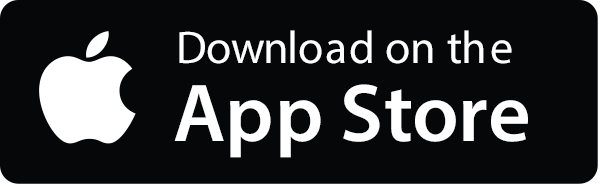
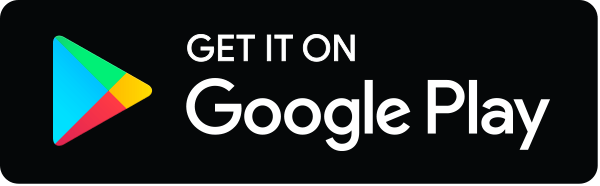