The term ‘autoinflammatory disease’ was first proposed in 1999 to encompass some of the distinct clinicopathologic features of a group of monogenic conditions, characterised by recurrent episodes of inflammation, without high-titre autoantibodies or antigen-specific T cells. It was subsequently observed that several of these conditions were caused by mutations in proteins involved in the innate immune response, including, among others, components of the NLRP3 inflammasome, cytokine receptors (tumour necrosis factor receptor 1 (TNFR1)) and receptor antagonists (interleukin 1 receptor antagonist (IL-1RA)). More recently, additional mechanisms linking innate immune-mediated inflammation with a variety of cellular processes, including protein misfolding, oxidative stress and mitochondrial dysfunction, have been recognised to play a role in the pathogenesis of some monogenic autoinflammatory conditions, and also in more common diseases such as type 2 diabetes (T2D), previously perceived as a metabolic disorder, but reclassified as a chronic inflammatory condition. NLRP3 inflammasome activation is induced by islet amyloid polypeptides (IAPPs) in T2D and this condition may, in future, be more commonly treated with targeted anti-cytokine therapies. Caspase 1 activation and release of IL-1β/IL-1 family members is central to the pathogenesis of many autoinflammatory syndromes, as evidenced by the effectiveness of anti-IL-1 biologics in treating these disorders. However, many patients continue to experience symptoms of chronic inflammation, and it will be necessary to translate discoveries on the immunopathology of these conditions into more effective therapies. For example, in tumour necrosis factor receptor-associated periodic fever syndrome (TRAPS), the pathogenesis may vary with each mutation and therefore future approaches to treatment of individual patients will require a more tailored approach based on genetic and functional studies.
Introduction
Discovery of periodic fever syndromes – early history of autoinflammation
The term ‘periodic disease’ was first employed by Hobart Reimann in 1948 to describe a clinical syndrome which manifested as benign paroxysmal peritonitis, periodic fevers, cyclical neutropenia and intermittent arthralgia . In 1958, Heller et al. introduced the designation ‘familial Mediterranean fever’ (FMF) for the syndrome described by Reimann, based on its increased prevalence in people of Mediterranean descent and characteristic clinical features . However, FMF, which usually has an autosomal recessive inheritance, is not restricted to these ethnic groups. Over subsequent years came recognition of the clinical aspects of other genetically determined recurrent fevers, both autosomal dominant and recessive, which were all collectively termed ‘hereditary periodic fevers (HPFs)’ ( Table 1 ).
Autoinflammation | Autoimmunity | |
---|---|---|
Immunological disruption | Innate immunity | Adaptive immunity |
Main cellular involvement | Neutrophils, macrophages | B and T cells |
Antibody involvement | Few or no autoantibodies | Autoantibodies present |
Clinical features | Recurrent, often seemingly unprovoked attacks | Continuous progression |
Conceptual understanding | Tissue-specific factors/danger signals | Breaking of self-tolerance |
Main genetic susceptibility | Cytokine and bacterial sensing pathways | MHC class II associations and adaptive response genes |
Therapy | Anti-cytokine (IL-1, TNF, IL-6) | Anti-B and T cell |
Examples | Monogenic hereditary periodic fevers, polygenic Crohn’s disease, spondylarthropathies | Monogenic ALPS and IPEX, polygenic RA and SLE |
The autosomal dominant conditions include tumour necrosis factor (TNF) receptor-associated periodic fever syndrome (TRAPS) (this condition was previously termed ‘familial Hibernian fever’ in 1982 ), familial cold autoinflammatory syndrome (FCAS), also known as familial cold urticaria (FCU), first described in 1940 , Muckle–Wells syndrome (MWS), characterised by urticaria, deafness and amyloidosis, described in 1962 and chronic infantile neurological cutaneous articular syndrome (CINCA; also known as neonatal-onset multisystemic inflammatory disease, abbreviated to NOMID) first described in 1981 . The three syndromes, MWS, FCAS and CINCA/NOMID, are all closely related as they share a number of clinical features and a common genetic basis. In 2001, a heterozygous mutation in the CIAS1/NLRP3 gene was found to be responsible for FCAS and MWS . A year later, a mutation in the same CIAS1/NLRP3 gene was reported to also cause CINCA/NOMID . Subsequently, the umbrella term ‘cryopyrin-associated periodic fever syndromes (CAPS)’ was introduced to highlight the common genetic basis of these conditions.
Hyperimmunoglobulinaemia D with periodic fever syndrome (HIDS) is an autosomal recessive HPF characterised by recurrent episodes of fever associated with lymphadenopathy, abdominal pain and skin rash, first reported in 1984 . The association of HIDS with homozygous mutations in the mevalonate kinase ( MVK ) gene was first described in 1999 .
With the identification of some of the genes underlying the HPFs, the term ‘autoinflammatory disease’ was first proposed in 1999 to encompass some of the distinct clinicopathologic features of these conditions, characterised by recurrent episodes of inflammation, without high-titre autoantibodies or antigen-specific T cells .
Autoinflammation – one end of immunological disease continuum
McGonagle and McDermott proposed in 2006 that the majority of inflammatory disorders are situated along an immunologic disease continuum (IDC), with genetic disorders of innate and adaptive immunity located at either end of the spectrum . HPFs are the prototypical genetically determined innate immune-mediated diseases, which may be associated with significant tissue destruction without evidence of adaptive immune responses and are designated as autoinflammatory due to their distinct immunopathological features ( Table 1 ).
There is increasing evidence that a combination of environmental, immunogenic and genetic aetiologies is instrumental in causing polygenic autoinflammatory and autoimmune diseases. Recognition of the central contribution of innate immune-related factors at target sites of disease has led to the idea of classifying some conditions (such as Behçet’s syndrome, psoriasis, psoriatic arthritis (PsA) and gout) as having major autoinflammatory components . Dysregulated innate immunity has been demonstrated in Crohn’s disease (CD), a polygenic disorder in which a breach in stability of the intestinal mucosal barrier defences causes abnormal handling of commensal luminal bacteria. CD has been classified as a polygenic autoinflammatory condition . Mutations in the NOD2 (NLRC2) gene encoding nucleotide-binding oligomerisation domain-containing protein 2 (NOD2)(NLRC2 protein), also known as caspase recruitment domain-containing protein 15 (CARD15 or IBD1), are present in about 20% of Caucasian patients with CD . The autophagy pathway has also been linked with CD through association with a coding single-nucleotide polymorphism (SNP) (T300A) in the ATG16L1 gene (chromosome 2q) . ATG16L1 encodes a protein involved in the autophagic mechanism, whereby intracellular bacteria are processed by lysosomal degradation; thus, a defect in this pathway may produce an inappropriate response to gut bacteria.
A number of studies have established the contribution of the interleukin (IL)-23 receptor gene ( IL23R ) to CD risk . The IL23R gene has also been associated with major histocompatibility complex (MHC) human leukocyte antigen (HLA) class I-related conditions, such as spondyloarthritis , psoriasis and Behçet’s disease . Although CD does not usually have HLA class I associations, a genetic overlap exists between CD and some MHC class I-associated diseases, including psoriasis .
These aforementioned inflammatory diseases exhibit dysregulated innate immunity and are genetically distinct from autoimmunity, but may demonstrate some evidence of adaptive immune responses . Classical autoimmune diseases, with autoantibody and MHC class II associations, including celiac disease and systemic lupus erythematosus (SLE), have adaptive immune genetic associations, including cytotoxic T-lymphocyte antigen-4 ( CTLA4 ) and protein tyrosine phosphatase, non-receptor type 22 ( PTPN22 ) that regulates some signalling pathways in T and B cells.
The proposed IDC classification is relevant to the clinical situation, because innate immune-mediated disorders respond better to cytokine antagonism whereas autoimmune-mediated diseases may respond to anti-T and B cell therapies. Furthermore, some conditions such as systemic juvenile idiopathic arthritis (sJIA) and ankylosing spondylitis (AS) have been reclassified as autoinflammatory diseases primarily based on response to IL-1 antagonism in the case of sJIA and innate immune system abnormalities in the case of AS .
Immunopathogenesis
Many paths lead to autoinflammation
Masters et al. have proposed a classification scheme for autoinflammatory disorders based on molecular mechanisms rather than clinical classification . They have defined six categories of autoinflammatory disease: IL-1β activation disorders (inflammasomopathies), nuclear factor (NF)-kappaB (NF-κB) activation syndromes, protein misfolding disorders, complement regulatory diseases, disturbances of cytokine signalling and macrophage activation syndromes. Therefore, in the 15 years that have elapsed since discovery of the genetic basis of FMF (the most prevalent HPF), the study of HPFs has evolved from the initial concept of autoinflammatory disease into novel classifications of these diseases with delineation of the central role of the innate immune system and the functional basis of some of these conditions. We explore several of these concepts and some newly proposed mechanisms in more detail.
Cytokine excess – NLRP3 inflammasome activation in the pathogenesis of CAPS
IL-1β is a potent pro-inflammatory cytokine, which is synthesised early in response to infection and tissue injury by cells of the innate immune system. It has multiple effects, including induction of fever and hepatic acute phase response, recruitment of neutrophils and induction of other pro-inflammatory cytokines, such as TNF and IL-6 . In excess, IL-1β can have detrimental physiological effects leading to tissue damage, bone reabsorption, collagen deposition and, in very large amounts, it can cause haemodynamic shock . Tight regulation of IL-1β synthesis and release is therefore essential to ensuring that the inflammatory response is of appropriate magnitude to deal with the threat posed by infection but, coincidentally, causing the least harm to the host. In part, this is achieved by having a number of different steps governing the synthesis, release and action of IL-1β.
IL-1β is first synthesised as an inactive 269-residue precursor (pro-IL-1β). Caspase-1 is a cysteine-protease that processes both pro-IL-1β and pro-IL-18 to generate the bioactive cytokines, IL-1β and IL-18, and to initiate pathogen-specific immune responses . The inactive pro-IL-1β form is found in monocytes, macrophages and dendritic cells, but only at very low levels in the absence of pro-inflammatory stimuli. Pro-IL-1β synthesis, or the priming step in IL-1β production, is induced by a number of pro-inflammatory pathways that recognise pathogen- or danger-associated molecular patterns (PAMPS and DAMPS, respectively)( Fig. 1 ). For example, lipopolysaccharide (LPS) activation of Toll-like receptor (TLR)4 and muramyl dipeptide (MDP) stimulation of NOD2 are two pathways that recognise a large number of the common Gram-negative and Gram-positive bacterial pathogens. Together with the pro-inflammatory cytokine, TNF, but also IL-1α and IL-1β itself, all of these pathways stimulate pro-IL-1β production by activating the transcription factor NF-κB .

The maturation of pro-IL-1β into the biologically active cytokine is dependent on the presence of secondary stimuli, which ultimately activate caspase-1 to cleave the immature cytokine into mature form, IL-1β. This process is dependent on various macromolecular platforms termed ‘inflammasomes’. The NLRP3 inflammasome is the most studied caspase-1-activating complex and the one which is mutated and functionally abnormal in CAPS. NLRP3 activation is itself a complex multi-stage process. It requires a priming step in monocytes, which is thought to increase the expression of NLRP3 in the target cells, in addition to usually requiring a second signal in macrophages, which may involve intracellular potassium (K + ) flux, adenosine triphosphate (ATP) and/or reactive oxygen species (ROS) ( Fig. 1 ). The importance of the second signal is discussed in some detail later.
So in the resting state, NLRP3 expression in cells is very low and the activation requires transcription of NLRP3, which can be induced by signals such as LPS, TNF and IL-1β that also promote the transcription of pro-IL-1β. The second signal is thought to be required for assembly of the NLRP3 inflammasome and also its activation in macrophages . NLRP3 protein contains a central NACHT or nucleotide-binding and oligomerisation domain, which has a role in assembly of the inflammasome. The majority of CAPS-causing mutations are found in the NACHT domain of NLRP3.
Early studies suggested that NLRP3 mutations in CAPS probably lead to gain of function as macrophages from MWS patients were found to produce significantly more IL-1β compared to healthy controls . However, the precise mechanisms responsible for this have not been fully worked out. One suggestion is that these mutations lead to spontaneous oligomerisation of the NLRP3 inflammasome subunits around mutated NACHT domain , possibly by removing an inhibitory loop, making the mutated inflammasome constitutively turned on and independent of the need for secondary signals for its activation. This leads to subsequent caspase-1 activation with excessive and inappropriate IL-1β release. This hypothesis is supported by observations that LPS alone is sufficient to cause caspase-1 activation in macrophages harbouring CAPS-causing mutations. Furthermore, cytosolic K+ that normally prevents activation of the NLRP3-inflammasome fails to do so if cells contain NLRP3-associated mutations .
Recently, a mouse model of CAPS was developed by two different groups, which recapitulated many concepts relevant to the pathogenesis of CAPS . They were able to demonstrate that inflammation in different tissues was mainly due to neutrophil infiltration and not dependent on B and T cells. In addition, the inflammatory response was also conditional on expression of the mutated NLRP3 in the cells of myeloid origin and excessive production of IL-1β. An interesting observation, which was also seen in CAPS patients, was IL-1β-dependent skewing of T-helper response towards Th17 phenotype. While IL-1β seems to be involved in polarisation towards the Th17 lineage, it also has a well described and strong impact on the production of the Th1 lymphokine interferon gamma (IFNγ) in activated cells . Indeed, the proliferation of all CD4 + T cell subsets may be enhanced by IL-1β . The exact relevance of these observations in the pathogenesis of CAPS is still unclear as the patients readily respond to IL-1 blockade. This latter observation offers further supporting evidence of the principal role that aberrant IL-1β release plays in the pathogenesis of CAPS. It is still unclear, however, which triggers precipitate attacks in all CAPS patients, how these triggers operate and why only certain tissues and organs are affected, and why some mutations are associated with a more severe clinical phenotype.
The importance of tight regulation of IL-1 activity is further illustrated by another autoinflammatory condition that shares some clinical features with the more severe spectrum of CAPS diseases (NOMID/CINCA) but has a somewhat different immunopathological basis. Deficiency of the IL-1 receptor antagonist (DIRA) is an autosomal recessive disease resulting from mutations in IL-1RN , the gene encoding the IL-1 receptor antagonist (IL-1Ra) . IL-1Ra is produced at the same time as IL-1α and IL-1β and limits the effects of these two cytokines on their common receptor. Interestingly, stromal cells (fibroblasts), which mainly express IL-1α (but not β), are also an important source of IL-1Ra and may make an important contribution to local tissue IL-1 activity control. We have recently shown that dermal fibroblasts are also significant producers of IL-18 binding protein (IL-18BP), another endogenous antagonist, but not IL-18 . This finding supports the emerging role of tissue-resident stomal cells as regulators of inflammatory responses. Unopposed action of IL-1 cytokines in DIRA results in a perinatal onset of skin pustulosis, joint swelling and various bone malformations, including painful osteolytic lesions, periostitis affecting the distal ribs and long bones and heterotopic bone formation . The somewhat different clinical phenotype seen in DIRA, in comparison with CINCA/NOMID, suggests the possibility of a greater role for IL-1α in disease pathogenesis. DIRA patients respond rapidly to anakinra, which is a synthetic IL-1 receptor antagonist (IL-Ra) .
The role of NLRP3 in the pathogenesis of CAPS is reasonably well established, with general agreement that gain-of-function mutations lead to the excessive IL-1β release. Inappropriate activation of the NLRP3 inflammasome and excessive IL-1β release has also been implicated in the pathogenesis of a number of other conditions, including T2D, where the role of chronic inflammation in disease pathogenesis has recently gained greater recognition. Here the accumulation of IAPP is thought to lead to inappropriate NLRP3 inflammasome activation and release of IL-1β with detrimental consequences for pancreatic β islet cells .
However, more recently, NLRP3 activation has been shown to have a protective role in two unrelated conditions, possibly through the induction of IL-18. In CD, reduced NLRP3 expression resulting from sequence changes in the promoter region has been associated with increased risk of developing the disease . The explanation for this association is not entirely resolved and likely to be quite complex. NLRP3 expression and activation has a protective role in the majority of animal models of induced colitis , which is contrary to the expectation that reduced NLRP3 expression, as demonstrated in monocytes of CD patients , might be expected to reduce inflammation in the bowel. However, this may not be entirely surprising since it is possible that appropriate NLRP3 activity is essential to maintain the immunological barrier to commensal gut micro-organisms. In addition, IL-18, whose maturation is also dependent on NLRP3 inflammasome, has been shown to have a specific role in promoting the repair of gut epithelium . Another line of evidence is based on the observation that active caspase-1 is involved in the non-conventional secretion of leaderless proteins – at least in epithelial cells which line all barrier organs . All members of the IL-1 family are leaderless proteins, and this is also true for important anti-inflammatory molecules, such as IL-37 . However, so far, experimental evidence for impaired secretion of anti-inflammatory mediators in patients with impaired caspase-1 activation is still lacking.
In the case of age-related macular degeneration (AMD), the roles of NLRP3 and IL-18 are somewhat controversial. NLRP3 (−/−) but not IL-1β (−/−) mice were found to be more susceptible to two different experimental models of AMD and drusen (focal extracellular deposits on the Bruch’s membrane below the retinal pigment epithelium (RPE) in the macula) isolated from donor AMD eyes caused activation of the NLRP3 inflammasome . Taken together, these findings suggest that drusen-induced NLRP3 activation is protective in AMD due to its role in IL-18 maturation. However, two subsequent publications suggest that NLRP3 activation due to oxidative stress in RPE cells , or resulting from Dicer1 loss or Alu RNA exposure, may have detrimental effects resulting in AMD .
Although the discussion so far has been mostly focussed on IL-1β release that is dependent on NLRP3 and caspase-1 activation, it is increasingly recognised that IL-1 processing and maturation can also occur independently of these mechanisms . This, in turn, might have specific implications when faced with disease states where an IL-1 signature is evident but the cause for such an observation is not obviously linked with inflammasomes or caspase-1 activation. A highly inflammatory disease to mention in this context is Netherton syndrome, which is characterised, among other symptoms, by severe eczema and universal pruritus (itch). Netherton syndrome is the result of a loss-of-function mutation in serine peptidase inhibitor, Kazal type 5 (SPINK5), which encodes for a serine protease inhibitor expressed in normal skin (lympho-epithelial Kazal-type-related inhibitor (LEKTI)) . High IL-18 expression has been demonstrated in the skin of these patients . Gout also has an IL-1-mediated autoinflammatory component, and activation of neutrophils in gout is associated with proinflammatory neutrophil extracellular trap formation (NET) and extracellular IL-1β production, which may be elastase mediated .
Gene dosage and the modifier effect – mediterranean fever (MEFV) mutations in FMF
Although the genetic cause of FMF has been known for over a decade, the exact explanation of how the mutated pyrin gene, also known as MEFV (mediterranean fever), causes inflammation remains incomplete. The fact that pyrin is predominantly expressed in cells of myeloid origin such as neutrophils, monocytes and dendritic cells and that FMF has an apparent autosomal recessive pattern of inheritance, have led to the assumption that the inflammation in FMF is caused by inappropriate activation of the innate immune system and the result of reduced or complete loss of pyrin function. Pyrin has a complex structure and expression pattern. The full-length human pyrin, which has four domains, an N-terminal pyrin domain (PYD), followed by two B-box zinc-finger and coiled-coil domains and a C-terminal B30.2 domain, is expressed in the cytoplasm, whilst the short forms, which are created by caspase-1-mediated cleavage, migrate to the nucleus. Various overexpression experiments have demonstrated that pyrin can interact with apoptosis-associated speck-like protein containing a caspase recruitment domain (CARD) (ASC) to limit NLRP3 activation , but it can also bind capsase-1, via the B30.2 domain , to negatively regulate the enzyme activity. This latter effect of pyrin is particularly interesting as it provides a plausible explanation for how these mutations may affect pyrin function since most pathogenic mutations are in this B30.2 domain . However, overexpression of pyrin has also been shown to induce caspase-1 activation in cells that stably express ASC . Furthermore, short-forms of pyrin were shown to promote NF-κB activation and transcription of pro-inflammatory cytokines, the effects of which were particularly enhanced in cells harbouring the mutated protein . In addition, mice expressing truncated forms of pyrin were found not to have a phenotype resembling FMF , whilst the majority of pathogenic MEFV mutations are missense changes and, to date, no null mutations have been identified . All these observations suggest that pyrin mutations in FMF result in gain rather than loss of protein function. Further support for this notion comes from recent work that was done in pyrin-deficient and ‘knock-in (KI)’ mice harbouring mutant human B30.2 domains, since the murine pyrin homologue does not contain B30.2 domain . Similarly, to mice expressing the truncated protein , pyrin-deficient animals and heterozygous KI mice have no clinical features of FMF, suggesting that loss of pyrin function alone is insufficient to recreate the pro-inflammatory phenotype . On the other hand, mice with two mutated copies of the gene showed a number of inflammatory features consistent with the human disease, including generalised neutrophilia, dermatitis and arthritis, but the hemizygous animals showed no evidence of the disease . Taken together, these findings suggest that disease-associated mutations lead to gain of pyrin function but that disease expression depends on the amount of mutated protein produced. This might explain why certain heterozygous pyrin mutations result in disease manifestation, albeit of a milder phenotype . Furthermore, this might also explain why MEFV sequence changes have been identified in chronic inflammatory diseases, such as Behçet’s disease , systemic AA amyloidosis and CD, , where they are thought to have pro-inflammatory disease-modifying effects.
The role of dysregulated unfolded protein response in the complex pathophysiology of TRAPS
A variety of mechanisms have been suggested to explain how autosomal dominant mutations of TNFRSF1A lead to the clinical manifestations of TRAPS ( Fig. 2 ). Nevertheless, the link between these mutations and associated phenotypes remains poorly understood with much apparent clinical heterogeneity between different mutations as well as among patients with the same mutation.

The reason for this might be due to the complexity of TNF signalling pathways, which are regulated on multiple levels and have a multitude of effects. Activation of tumour necrosis factor receptor 1 (TNFR1), for example, can lead to activation of NF-κB and the mitogen-activated protein kinase (MAPK) cascade , which will result in either cell survival, or induction of an inflammatory response. Alternatively, activation of caspase-3 will eventually lead to cell death due to apoptosis . The myriad of often conflicting effects mediated by TNFR1 is possible because the intracellular domain of the receptor can engage with several different intracellular pathways via the TNFR-associated death domain protein (TRADD)-containing macromolecular platform . TNF is mainly produced by epithelial and innate immune cells, including macrophages, monocytes, neutrophils and NK cells, but also by some T-cell subsets. TNFR1 is expressed as a homotrimer on the cell surface and activates TNFR1 and TNFR2, with both receptors also forming homotrimers following engagement of the membrane-bound form of TNF (mTNF). In addition, TNFR1 can recognise a soluble form of TNF (sTNF), released from the cell membrane by the TNF-α converting enzyme (TACE/a disintegrin and metalloprotease domain 17 ADAM17) . This same enzyme also cleaves TNFR1 and TNFR2 from the cell surface, and these soluble forms of receptors are thought to have a predominantly neutralising effect on TNF .
Early studies into the pathogenesis of TRAPS suggested that mutated TNFR1 might be resistant to this enzymatic cleavage, resulting in impaired clearance of TNFR1 from the cell membrane . This was an attractive hypothesis as it offered an explanation why certain patients had low levels of soluble TNFR1 (sTNFR1) in patients’ serum between attacks. It could also possibly explain why the pathogenic mutations were either missense, small deletions or insertions found in the extracellular domains, as these may affect the secondary structure and therefore limit access to the proteolytic cleavage site. However, the TNFR1 shedding defect is not a universally reproducible finding in all patients with TRAPS , whilst treatment with etanercept, which is a fusion molecule of TNFR2/FcIg, designed to mop up soluble TNF, is only partially effective .
Increased NF-κB activation has also been associated with some mutations, and, more recently, additional mechanisms have been suggested to explain the pro-inflammatory effects of mutated TNFR1. Simon et al. reported that TRAPS patients demonstrated higher MAPK activation in response to bacterial LPS, a TLR4 ligand, than healthy controls and also showed a hyper-responsiveness to LPS whereby TNFRSF1A mutant peripheral blood mononuclear cell (PBMC) could respond to low dose LPS (0.01 ng ml −1 ) . Subsequently, it was shown that this might in part be due to the effects of ROS on MAPK activation . Monocytes and neutrophils from TRAPS patients showed higher baseline levels of ROS than cells from healthy donors. Increased ROS was shown to mediate increased pro-inflammatory cytokine secretion and also increased MAPK activation, which is therefore thought to be mediating the cytokine release. ROS are known to inactivate MAPK phosphatases by oxidation of the catalytic cysteine residue, thereby enhancing MAPK activation. Somewhat surprisingly, further studies demonstrated the source of ROS production to be the mitochondria, mainly because of enhanced ROS production rather than blocked mitophagy, which was recently implicated in the pathogenesis of other autoinflammatory conditions such as CD .
Another important observation about the pathogenesis of TRAPS is that trafficking of the mutated receptor to the cell surface appears to be impaired . Low surface expression of TNFR1 has been reported in patients with TRAPS , and furthermore, retained aggregates of TRAPS-associated mutant protein have been reported in the endoplasmic reticulum (ER) of transfected cells . Although earlier studies reported no clear evidence of ER stress associated with this phenomenon , we recently investigated the possible convergence of ER stress pathways and enhanced ROS production in TRAPS patients . We hypothesised that retention of the mutated receptor within the ER would cause a degree of ER stress and that this would facilitate activation of inflammatory pathways, including enhanced cytokine release and ROS generation, and could confer the reported hyper-responsiveness to LPS. We found that resting monocytes from TRAPS patients showed increased expression of protein kinase-like ER kinase (PERK) and spliced X-box binding protein 1 (sXBP1), which are two markers of ER stress and activated as part of the unfolded protein response (UPR) . However, these findings were not associated with other markers of ER stress, as we found normal expression levels of downstream target genes, such as synoviolin and DNA-damage-inducible transcript 3. Furthermore, we confirmed that TRAPS cells showed increased baseline total and mitochondrial-specific ROS production , which was increased further in response to IL-6 stimulation. We also found that LPS promoted sXBP1 activation, which was abolished by the addition of antioxidants . The importance of sXBP1 in TLR4 signalling was previously demonstrated by Martinon et al. who showed that sXBP1 is generated in response to LPS independently of ER stress, as other UPR pathways were not activated . The sXBP1 was essential for sustained pro-inflammatory cytokine production, and mild ER stress could also greatly facilitate production of inflammatory cytokines, such as TNF and IL-6, as sXBP1 was found to bind directly to the promoter region of these cytokines . Interestingly, sXBP1 was also recently linked with the p38 MAPK signalling pathway; Lee et al. reported that p38 was able to directly phosphorylate sXBP1 leading to enhanced nuclear translocation and transcriptional activity . Therefore, sXBP1 can explain some of the hyper-responsiveness to LPS in TRAPS, and this might be enhanced further by the effect that p38 MAPK pathway activation has on sXBP1 stability.
The link between ER and oxidative stress, although previously established, in this situation is, however, less clear. XBP1 does also have a role in oxidative stress responses since a number of gene targets of XBP1 are known to have roles in redox homeostasis and cell survival during hypoxic conditions. Interestingly, it is the unspliced form of XBP1 (uXBP1), which was found to induce expression of antioxidant genes , including catalase, superoxide dismutase and thioredoxin. This role was independent of sXBP1, as the spliced isoform was unable to regulate the expression levels of these antioxidants. We found that the uXBP1 transcript levels were not significantly elevated in TRAPS patients when compared to HC, despite the increased ROS levels present in the patients’ cells . These findings suggest a possible defect in the antioxidative responses in TRAPS patients.
Lastly, the murine models of TRAPS suggest that expression of both the wild type and mutant receptor is necessary to replicate the inflammatory phenotype of TRAPS. Mice homozygous for TRAPS-associated TNFR1 mutants, rather than suffering from spontaneous inflammatory problems, show resistance to LPS-induced septic shock , which is a phenotype previously described in TNFR1-deficient animals. This is in keeping with the disease model whereby retention of the mutated receptor within the ER provides a low-grade stimulus, on which other signals, including TNF itself (due to the presence of wild-type receptor), act to induce the hyper-inflammatory state typical of TRAPS.
Novel mechanisms of autoinflammation – mutated immunoproteasome
Proteasome-associated autoinflammatory syndromes is a term that has been recently introduced to highlight the common genetic basis of a number of rare autoinflammatory conditions with overlapping clinical features . Hypomorphic mutations affecting the inducible β5i subunit of the PSMB8 proteasome (proteasome subunit β type 8) have been reported in patients with chronic atypical neutrophilic dermatosis with lipodystrophy and elevated temperature (CANDLE), joint contractures, muscle atrophy, microcytic anaemia and panniculitis-induced childhood-onset lipodystrophy . (JMP), Nakajo-Nishimura syndrome (NNS) and Japanese autoinflammatory syndrome with lipodystrophy (JASL).
The proteasome is an evolutionarily conserved cylindrical organelle which has an essential role in protein degradation. It is composed of two α and two β rings which combine to give it its cylindrical shape ( Fig. 1 ). The α rings have a role in substrate capture whilst the proteolytic function is confined to the β rings. Each ring is composed of seven individual proteins; the inner two rings are made of seven β subunits that contain three to seven protease active sites. The proteins destined for degradation are usually tagged with polyubiqutin chains, a process that is used in regulating many cellular functions including activation of NF-κB. Of particular interest is the role of the proteasome in antigen processing during infection. Pathogen-derived proteins are degraded by the proteasome for presentation by MHC class I molecules at the cell surface. However, this process is much more efficient and the degradation products fit the groove of the MHC class I molecules better when several subunits of the β ring are replaced by the inducible (i) subunits β1i, β2i and β5i. The resulting so-called immunoproteasome, assembled with these alternative subunits, is highly expressed in haemopoietic cells and expression of the (i) subunits is directed by type I interferons. Recent data from animal psmb8/lmp7 KO studies suggest that the immunoproteasome also plays a part in maintaining cell homeostasis by removing damaged proteins accumulated as a result of various cellular processes including oxidative stress.
In silico analysis of the mutated β5i subunits found in CANDLE and JMP syndromes suggest that formation of the immunoproteasome is impaired in these conditions . Agrawal et al. showed that the proteasome from JMP patients had markedly reduced chymotrypsin-like activity but preservation of trypsin and peptidyl glutamyl peptide-hydrolysing function, which is in keeping with specifically impaired activity of the immunoproteasome . The same patients were found to have significantly elevated IFNγ and IL-6 with elevated erythrocyte sedimentation rate (ESR) and serum γ globulins, but not elevated TNF or IL-1β. This particular cytokine signature seems to be typical of impaired immunoproteasome function, since patients with CANDLE syndrome were also found to have elevated IFNγ and IL-6, but not TNF. These patients also had significantly elevated levels of IFNγ-inducible protein 10 (IP-10 or C-X-C motif chemokine 1 CXCL10), and elevated levels of monocyte chemotactic protein 1 (MCP-1 or Chemokine (C-C motif) ligand 2 CCL2) and regulated upon activation, normal T-cell expressed, and secreted (RANTES or CCL5). Further evidence that excessive IFNγ signalling might play a role in the pathogenesis of this condition came from gene expression studies of the whole blood and from analysis of signal transducer and activator of transcription-1 (STAT-1) signalling pathway in monocytes. The IFNγ pathway was demonstrated to be the most differentially regulated, whilst, at the same time, monocytes from CANDLE syndrome patients showed higher STAT-1 phosphorylation in response to IFNγ compared to healthy controls and patients with CINCA/NOMID .
Based on these findings, it has been proposed that the ongoing IFNγ stimulation in patients with CANDLE syndrome and related conditions results from inability of the immunoproteasome to deal appropriately with accumulation of damaged proteins leading to chronic cellular stress. As a result, the cellular stress remains unresolved and IFNγ stimulation continues unabated. In keeping with this hypothesis is the observation that disease flares are associated with infections and other stressful events. Furthermore, affected tissues such as muscle and fat showed inclusion bodies which might suggest accumulation of oxidant-damaged/aggregated proteins leading to apoptosis.
Interestingly, lipodystrophy, which is a recognised complication of protease inhibitors (PIs), a class of anti-human immunodeficiency virus (HIV) medication, might be caused by modulatory effects of these agents on the proteasome. Ritonavir, for example, was shown to specifically inhibit the chymotrypsin-like activity, whilst enhancing trypsin-like activity of the proteasome . Although this effect was originally thought to modulate antigen processing only, in the light of our current understanding of how chymotrypsin-like activity is affected in the mutated immunoproteasome, it is possible to speculate that this might be one of the mechanisms which is responsible for PI-induced lipodystrophy.
Autoinflammation of the skin; IL-36 and deficiency of IL-36 receptor antagonist (DITRA) and CARD14 in psoriasis susceptibility locus 2 (PSORS2)
A common feature of all IL-1 family members is the tight control of their released active forms and the presence of soluble antagonists; for example, IL-1α/β are controlled by the endogenous antagonist IL-1RA, IL-18 by IL-18 binding protein (IL-18BP) and IL-36 molecules are controlled by IL-36 receptor antagonist (IL-36RA, former name IL-1F5), which shows a mode of action comparable to IL-1RA. IL-36 has three different subtypes, IL-36α (IL-1F6), IL-36β (IL-1F8) and IL-36γ (IL-1F9). These homologous cytokines all bind to the same receptor which consists of IL-1RAcP (also shared by IL-1α,β and IL-33) and IL-1Rrp2. Therefore, IL-36RA, which competes with these cytokines for IL-1Rrp2 receptor binding and prevents recruitment of IL-1RAcP, balances the functional activity of all IL-36 members.
Recently, loss-of-function mutations of the IL-36RA have been associated with severe pustular psoriasis, as described by two independent groups . The name DITRA has been proposed for this autosomal recessive autoinflammatory condition. All IL-36 molecules, including the IL-36RA, show high biological activity only after cleavage ; the responsible endogenous protease, however, has not been identified so far.
Recent data suggest that IL-1 family members are intimately involved in the cellular response of tissue cells characterising psoriatic inflammation. IL-36 has been identified as one of the most highly expressed genes in lesional as compared to non-lesional psoriasis .
We have previously shown that cultured skin cells derived from psoriasis patients show increased expression of IL-36 upon cytokine stimulation , which is not paralleled by IL-36RA induction. The intrinsic difference in the expression level of IL-36α/γ between cultured and passaged cells derived from psoriasis or healthy individuals is dramatic. IL-36 has not been identified as a genetic susceptibility locus in psoriasis genome wide association studies (GWAS). Therefore, the observed difference could be due to genetic variants in signalling pathways, as suggested by a recent study of gain-of-function variants in CARD14 , post-translational regulators or epigenetic in nature. Data for PsA are not yet available as IL-36 has only recently come into the picture for psoriatic inflammation research . The best-described inducer of IL-36 messenger RNA (mRNA) expression is the synergistic action of TNF and IL-17 , which are both up-regulated in psoriasis and PsA and blockade of which improve clinical symptoms.
Interestingly, PsA and skin psoriasis share common genetic variants that are important for the NF-κB-related pathways (e.g., A20 [TNFAIP3], TNIP1 and TRAF3IP2) . Therefore, psoriatic disease may fit into the proposed novel classification as NF-κB-related autoinflammatory condition.
In the early 1990s, GWAS undertaken to find psoriasis-susceptibility genes provided evidence for the presence of a disease locus within the MHC on chromosome 6p21.3, referred to as PSORS1 ( psoriasis susceptibility 1 ) (for review: ). It was later suggested that HLA-C was the most likely PSORS1 candidate gene . With regard to a second psoriasis susceptibility site called PSORS2, a recent study revealed CARD14 gain-of-function mutations as ‘responsible’ for the PSORS2 findings . CARD14 activates NF-κB and compared with wild-type, the mutated form leads to increased activity of NF-κB and up-regulation of a subset of psoriasis-associated mediators in skin-resident cells. This is the point at which the circle closes as we have come back to the above described IL-36, which is indeed among the mediators found to be up-regulated in cells with mutated CARD14 , along with IL-8, which attracts neutrophils, and CCL20 which attracts immature dendritic and Th17 cells into the psoriatic lesions. This study thus further supports the notion that psoriasis may fit very well into the ‘NF-κB’ pathway subcategory.
Integration of NLRP3, ER stress and mitochondria-mediated inflammatory responses
Although the close link between metabolic stress and inflammation has long been suspected, it is only recently that our understanding of the molecular mechanisms that underpin this relationship has significantly advanced ( Fig. 1 ). The three key areas of research that have mostly contributed to this are: NLRP3 activation and the role of this process in the pathogenesis of conditions such as T2D, the relationship between ER stress and generation of inflammatory cytokines and the role of the mitochondria in innate immune responses, specifically mitochondrial-derived ROS (mROS).
NLRP3 inflammasome activation is a complex process and has been extensively investigated over the last several years. Although many potential activators of the inflammasome have been identified, questions have been asked how such diverse stimuli can all cause activation of the same macromolecular complex. The multistage activation of the NLRP3 inflammasome was proposed to explain some of the findings, with agreement that the first stage involves up-regulation of NLRP3 expression as a result of, for example, TLR stimulation. There has been much more controversy about what processes govern the assembly of NLRP3 inflammasome and ultimately caspase-1 activation. This field of research was led by the late Jurg Tschopp who proposed three different, mutually non-exclusive, models of NLRP3 activation: the channel model, the lysosome rupture model and finally the ROS model . The channel model explains the need for the ATP and K+ flux, whereby activation of P2X7, an ATP-gated ion channel, triggers rapid K+ efflux from the cell, which is an absolute requirement in most proposed models of NLRP3 activation. In addition, this leads to pore formation in the cell membrane as a result of recruitment of the pannexin 1 hemi-channel , which allows PAMPs such as MDP to enter the cell and engage NLRP3. Although pore-forming microorganisms such as α-toxin-producing Staphylococcus aureus are potent activators of the inflammasome , this could result from allowing K+ flux through the pore alone, rather than direct binding of the NLRP3, which, so far, has not been demonstrated for most of the known activators.
The lysosome rupture model suggests that the lysosomal protein cathepsin B, released after lysosomal rupture, triggers NLRP3 activation. This would explain how large particulate activators, such as alum and silica can activate the NLRP3 inflammasome . Cathepsin B inhibition does impair NLRP3 activation in human cells , but similar findings have not been consistently replicated in cathepsin B KO mouse models .
The usual sources of endogenous ROS are nicotinamide adenine dinucleotide phosphate (NADPH) oxidases (NOX), which are active in phagosomes, the Erol-1DPI oxidative folding system in the ER and the mitochondrial electron transport chain (ETC). It would therefore make sense if NLRP3 activation was governed by changes in ROS, as this would allow inflammasome activation in response to a variety of important cellular stresses. NLRP3 was found to associate with thioredoxin-interacting protein (TXNIP; also known as VDuP1) after cells were treated with NLRP3 activators . This is an ROS-dependent process, since in unstimulated cells TXNIP is constitutively bound to and inhibited by the oxidoreductase, thioredoxin. As a result of increases in cellular ROS concentration, TXNIP dissociates from thioredoxin and binds to NLRP3. This model is supported by observations that KO or knockdown by small interfering RNA (siRNA) of TXNIP leads to reduced IL-1β secretion, whilst the knockdown of thioredoxin enhances NLRP3 activation . Furthermore, pharmacological blockade of ROS has also been shown to reduce inflammasome activation .
Although ROS appear to be important activators of the NLRP3 inflammasome, the source of such ROS has been debated. NLRP3 inflammasome activation is not impaired in patients with chronic granulomatous disease (CGD), who are deficient in one of the NOX components . Traditionally, mROS was not regarded to have an important role in innate immune responses. However, more recently, mROS has been seen as a much more likely candidate after the discovery that it can be generated in response to bacteria in a TLR-dependent fashion. Coupling of TLR1/2/4 signalling to mitochondrial complex I was demonstrated via TNF receptor-associated factor 6 (TRAF6), an evolutionarily conserved signalling intermediate in Toll pathways (ECSIT) . As already mentioned, mROS have also been particularly implicated in the pathogenesis of TRAPS . The role of mROS in NLRP3 activation has been shown more specifically by Zhou et al. who not only demonstrated inhibition of NLRP3 activation by disrupting mROS generation but also the physical proximity between the activated NLRP3 inflammasome and mitochondria. In resting cells, NLRP3 localises to the ER structures, but in the activated state, NLRP3 and ASC, an adaptor protein of the NLRP3 inflammasome, have a peri-nuclear location and co-localise with ER and mitochondria organelle clusters . It is perhaps not surprising that mROS is possibly one of the main activators of the NLRP3 inflammasome, since it is produced in response to many cellular stresses, including increased metabolic rate, hypoxia or membrane damage, which, in turn, places NLRP3 inflammasome activation at the centre of many important cellular responses.
The connection between NLRP3 and cellular stress does not stop there since activation of NLRP3 was recently shown to occur in response to ER stress independently of UPR pathways . The ER stress-triggered UPR has traditionally been associated with accumulation of misfolded proteins within the ER and attempts by the cell to rectify this problem. However, ER stress and components of the UPR pathway also induce inflammatory responses, through several distinct mechanisms. inositol-requiring enzyme 1 (IRE1) and PERK can activate the NF-κB, which is essential for initiating inflammation through the induction of a number of pro-inflammatory genes. These pathways converge to reduce availability of the inhibitor of NF-κB, IκB. In the case of the PERK pathway, this is due to its effect on global protein synthesis through the activation of eukaryotic initiation factor 2α (eIF2α), which appears to affect IκB more than NF-κB, as the former has a shorter half-life . IRE1 achieves NF-κB activation through its association with the adaptor protein TRAF2. The IRE1-TRAF2 complex can recruit IκB, which is then phosphorylated and degraded, resulting in nuclear translocation of NF-κB . Furthermore, this complex also activates c-Jun N-terminal kinases (JNKs), which in turn phosphorylates transcription factor activator protein 1 (AP1) . The latter, similarly to NF-κB, induces transcription of pro-inflammatory genes. NF-κB activation has also been linked to ROS and oxidative stress, generated as a result of an increased protein load on the ER and the demand for additional disulfide bond formation. Although the mechanism(s) involved is poorly understood, the observation that NF-κB activation can be reduced by antioxidants and calcium chelators supports this claim . This last observation might be particularly relevant to NLRP3 activation associated with ER stress, which is also dependent on the presence of ROS.
This interplay between NLRP3 activation, mROS and ER stress provides an explanation of how multiple mechanisms involving metabolic stress, innate immune activation and production of inflammatory cytokines, come together in the pathogenesis of some common chronic inflammatory conditions, considered to be autoinflammatory in nature. Examples include spondyloarthropathies, such as AS, inflammatory bowel disease, such as CD, T2D and arteriosclerosis, to name just a few amongst an ever increasing list.
AS was one of the first inflammatory conditions where this link to UPR was investigated. HLA-B27, which is an AS susceptibility locus, is prone to misfolding within the ER . B27/Huβ 2 m-transgenic rats, that overexpress the human HLA-B27 gene, develop arthropathy, psoriasis-like skin lesions and gastrointestinal inflammation . There is circumstantial evidence that macrophages from these animals, when stimulated with IFNα, IFNβ or TNF, demonstrate a more active UPR compared to controls . This observation was made on the basis of elevated BiP and sXBP1 mRNA levels in bone marrow-derived macrophages from these animals. More recently, UPR induced either by HLA-B27 misfolding or by pharmacological agents, was shown to act synergistically with LPS to induce macrophages from these animals to produce IL-23 . Furthermore, elevated IL-23 and IL-17 transcript levels were found in CD11 + antigen presenting cells (APC) and in CD4+ T cells, respectively, from the colonic lamina propria of the transgenic animals . The form of colitis usually seen in these animals was associated with a sixfold expansion of Th17 cells. However, here is evidence that IL-17 in spondyloarthritis is secreted by innate immune cells rather than T cells in the facet joints of patients with spondyloarthritis .
NLRP3 activation and ER stress have both been implicated in the pathogenesis of arteriosclerosis. Here, the accumulation of free cholesterol in the ER membrane leads to Ca 2+ release, activation of UPR and CCAAT/-enhancer-binding protein homologous protein (CHOP)-induced apoptosis . Several inflammatory pathways, including NF-κB, JNK, p38 and ERK1 and 2 (extracellular-signal-regulated kinase) are activated by loading macrophages with free cholesterol, with IRE1 and PERK potentially playing a role in NF-κB and JNK activation . Furthermore, oxidised lipids also induce UPR in human aortic endothelial cells . Finally, in vitro studies have linked ER stress-induction of activating transcription factor 4(ATF4) and sXBP1 with the production of inflammatory cytokine IL-6 and the IL-8 and CXCL3 chemokines. This was demonstrated in human aortic endothelial cells at baseline and as a result of the accumulation of oxidised lipids . More recently, cholesterol crystals have been shown to activate the NLRP3 inflammasome and release IL-1β in mouse and human macrophages .
It is beyond the scope of this chapter to discuss the pathogenesis of all the conditions mentioned here but many excellent reviews have recently been written on this subject and readers are encouraged to consult these for further information.
Immunopathogenesis
Many paths lead to autoinflammation
Masters et al. have proposed a classification scheme for autoinflammatory disorders based on molecular mechanisms rather than clinical classification . They have defined six categories of autoinflammatory disease: IL-1β activation disorders (inflammasomopathies), nuclear factor (NF)-kappaB (NF-κB) activation syndromes, protein misfolding disorders, complement regulatory diseases, disturbances of cytokine signalling and macrophage activation syndromes. Therefore, in the 15 years that have elapsed since discovery of the genetic basis of FMF (the most prevalent HPF), the study of HPFs has evolved from the initial concept of autoinflammatory disease into novel classifications of these diseases with delineation of the central role of the innate immune system and the functional basis of some of these conditions. We explore several of these concepts and some newly proposed mechanisms in more detail.
Cytokine excess – NLRP3 inflammasome activation in the pathogenesis of CAPS
IL-1β is a potent pro-inflammatory cytokine, which is synthesised early in response to infection and tissue injury by cells of the innate immune system. It has multiple effects, including induction of fever and hepatic acute phase response, recruitment of neutrophils and induction of other pro-inflammatory cytokines, such as TNF and IL-6 . In excess, IL-1β can have detrimental physiological effects leading to tissue damage, bone reabsorption, collagen deposition and, in very large amounts, it can cause haemodynamic shock . Tight regulation of IL-1β synthesis and release is therefore essential to ensuring that the inflammatory response is of appropriate magnitude to deal with the threat posed by infection but, coincidentally, causing the least harm to the host. In part, this is achieved by having a number of different steps governing the synthesis, release and action of IL-1β.
IL-1β is first synthesised as an inactive 269-residue precursor (pro-IL-1β). Caspase-1 is a cysteine-protease that processes both pro-IL-1β and pro-IL-18 to generate the bioactive cytokines, IL-1β and IL-18, and to initiate pathogen-specific immune responses . The inactive pro-IL-1β form is found in monocytes, macrophages and dendritic cells, but only at very low levels in the absence of pro-inflammatory stimuli. Pro-IL-1β synthesis, or the priming step in IL-1β production, is induced by a number of pro-inflammatory pathways that recognise pathogen- or danger-associated molecular patterns (PAMPS and DAMPS, respectively)( Fig. 1 ). For example, lipopolysaccharide (LPS) activation of Toll-like receptor (TLR)4 and muramyl dipeptide (MDP) stimulation of NOD2 are two pathways that recognise a large number of the common Gram-negative and Gram-positive bacterial pathogens. Together with the pro-inflammatory cytokine, TNF, but also IL-1α and IL-1β itself, all of these pathways stimulate pro-IL-1β production by activating the transcription factor NF-κB .
The maturation of pro-IL-1β into the biologically active cytokine is dependent on the presence of secondary stimuli, which ultimately activate caspase-1 to cleave the immature cytokine into mature form, IL-1β. This process is dependent on various macromolecular platforms termed ‘inflammasomes’. The NLRP3 inflammasome is the most studied caspase-1-activating complex and the one which is mutated and functionally abnormal in CAPS. NLRP3 activation is itself a complex multi-stage process. It requires a priming step in monocytes, which is thought to increase the expression of NLRP3 in the target cells, in addition to usually requiring a second signal in macrophages, which may involve intracellular potassium (K + ) flux, adenosine triphosphate (ATP) and/or reactive oxygen species (ROS) ( Fig. 1 ). The importance of the second signal is discussed in some detail later.
So in the resting state, NLRP3 expression in cells is very low and the activation requires transcription of NLRP3, which can be induced by signals such as LPS, TNF and IL-1β that also promote the transcription of pro-IL-1β. The second signal is thought to be required for assembly of the NLRP3 inflammasome and also its activation in macrophages . NLRP3 protein contains a central NACHT or nucleotide-binding and oligomerisation domain, which has a role in assembly of the inflammasome. The majority of CAPS-causing mutations are found in the NACHT domain of NLRP3.
Early studies suggested that NLRP3 mutations in CAPS probably lead to gain of function as macrophages from MWS patients were found to produce significantly more IL-1β compared to healthy controls . However, the precise mechanisms responsible for this have not been fully worked out. One suggestion is that these mutations lead to spontaneous oligomerisation of the NLRP3 inflammasome subunits around mutated NACHT domain , possibly by removing an inhibitory loop, making the mutated inflammasome constitutively turned on and independent of the need for secondary signals for its activation. This leads to subsequent caspase-1 activation with excessive and inappropriate IL-1β release. This hypothesis is supported by observations that LPS alone is sufficient to cause caspase-1 activation in macrophages harbouring CAPS-causing mutations. Furthermore, cytosolic K+ that normally prevents activation of the NLRP3-inflammasome fails to do so if cells contain NLRP3-associated mutations .
Recently, a mouse model of CAPS was developed by two different groups, which recapitulated many concepts relevant to the pathogenesis of CAPS . They were able to demonstrate that inflammation in different tissues was mainly due to neutrophil infiltration and not dependent on B and T cells. In addition, the inflammatory response was also conditional on expression of the mutated NLRP3 in the cells of myeloid origin and excessive production of IL-1β. An interesting observation, which was also seen in CAPS patients, was IL-1β-dependent skewing of T-helper response towards Th17 phenotype. While IL-1β seems to be involved in polarisation towards the Th17 lineage, it also has a well described and strong impact on the production of the Th1 lymphokine interferon gamma (IFNγ) in activated cells . Indeed, the proliferation of all CD4 + T cell subsets may be enhanced by IL-1β . The exact relevance of these observations in the pathogenesis of CAPS is still unclear as the patients readily respond to IL-1 blockade. This latter observation offers further supporting evidence of the principal role that aberrant IL-1β release plays in the pathogenesis of CAPS. It is still unclear, however, which triggers precipitate attacks in all CAPS patients, how these triggers operate and why only certain tissues and organs are affected, and why some mutations are associated with a more severe clinical phenotype.
The importance of tight regulation of IL-1 activity is further illustrated by another autoinflammatory condition that shares some clinical features with the more severe spectrum of CAPS diseases (NOMID/CINCA) but has a somewhat different immunopathological basis. Deficiency of the IL-1 receptor antagonist (DIRA) is an autosomal recessive disease resulting from mutations in IL-1RN , the gene encoding the IL-1 receptor antagonist (IL-1Ra) . IL-1Ra is produced at the same time as IL-1α and IL-1β and limits the effects of these two cytokines on their common receptor. Interestingly, stromal cells (fibroblasts), which mainly express IL-1α (but not β), are also an important source of IL-1Ra and may make an important contribution to local tissue IL-1 activity control. We have recently shown that dermal fibroblasts are also significant producers of IL-18 binding protein (IL-18BP), another endogenous antagonist, but not IL-18 . This finding supports the emerging role of tissue-resident stomal cells as regulators of inflammatory responses. Unopposed action of IL-1 cytokines in DIRA results in a perinatal onset of skin pustulosis, joint swelling and various bone malformations, including painful osteolytic lesions, periostitis affecting the distal ribs and long bones and heterotopic bone formation . The somewhat different clinical phenotype seen in DIRA, in comparison with CINCA/NOMID, suggests the possibility of a greater role for IL-1α in disease pathogenesis. DIRA patients respond rapidly to anakinra, which is a synthetic IL-1 receptor antagonist (IL-Ra) .
The role of NLRP3 in the pathogenesis of CAPS is reasonably well established, with general agreement that gain-of-function mutations lead to the excessive IL-1β release. Inappropriate activation of the NLRP3 inflammasome and excessive IL-1β release has also been implicated in the pathogenesis of a number of other conditions, including T2D, where the role of chronic inflammation in disease pathogenesis has recently gained greater recognition. Here the accumulation of IAPP is thought to lead to inappropriate NLRP3 inflammasome activation and release of IL-1β with detrimental consequences for pancreatic β islet cells .
However, more recently, NLRP3 activation has been shown to have a protective role in two unrelated conditions, possibly through the induction of IL-18. In CD, reduced NLRP3 expression resulting from sequence changes in the promoter region has been associated with increased risk of developing the disease . The explanation for this association is not entirely resolved and likely to be quite complex. NLRP3 expression and activation has a protective role in the majority of animal models of induced colitis , which is contrary to the expectation that reduced NLRP3 expression, as demonstrated in monocytes of CD patients , might be expected to reduce inflammation in the bowel. However, this may not be entirely surprising since it is possible that appropriate NLRP3 activity is essential to maintain the immunological barrier to commensal gut micro-organisms. In addition, IL-18, whose maturation is also dependent on NLRP3 inflammasome, has been shown to have a specific role in promoting the repair of gut epithelium . Another line of evidence is based on the observation that active caspase-1 is involved in the non-conventional secretion of leaderless proteins – at least in epithelial cells which line all barrier organs . All members of the IL-1 family are leaderless proteins, and this is also true for important anti-inflammatory molecules, such as IL-37 . However, so far, experimental evidence for impaired secretion of anti-inflammatory mediators in patients with impaired caspase-1 activation is still lacking.
In the case of age-related macular degeneration (AMD), the roles of NLRP3 and IL-18 are somewhat controversial. NLRP3 (−/−) but not IL-1β (−/−) mice were found to be more susceptible to two different experimental models of AMD and drusen (focal extracellular deposits on the Bruch’s membrane below the retinal pigment epithelium (RPE) in the macula) isolated from donor AMD eyes caused activation of the NLRP3 inflammasome . Taken together, these findings suggest that drusen-induced NLRP3 activation is protective in AMD due to its role in IL-18 maturation. However, two subsequent publications suggest that NLRP3 activation due to oxidative stress in RPE cells , or resulting from Dicer1 loss or Alu RNA exposure, may have detrimental effects resulting in AMD .
Although the discussion so far has been mostly focussed on IL-1β release that is dependent on NLRP3 and caspase-1 activation, it is increasingly recognised that IL-1 processing and maturation can also occur independently of these mechanisms . This, in turn, might have specific implications when faced with disease states where an IL-1 signature is evident but the cause for such an observation is not obviously linked with inflammasomes or caspase-1 activation. A highly inflammatory disease to mention in this context is Netherton syndrome, which is characterised, among other symptoms, by severe eczema and universal pruritus (itch). Netherton syndrome is the result of a loss-of-function mutation in serine peptidase inhibitor, Kazal type 5 (SPINK5), which encodes for a serine protease inhibitor expressed in normal skin (lympho-epithelial Kazal-type-related inhibitor (LEKTI)) . High IL-18 expression has been demonstrated in the skin of these patients . Gout also has an IL-1-mediated autoinflammatory component, and activation of neutrophils in gout is associated with proinflammatory neutrophil extracellular trap formation (NET) and extracellular IL-1β production, which may be elastase mediated .
Gene dosage and the modifier effect – mediterranean fever (MEFV) mutations in FMF
Although the genetic cause of FMF has been known for over a decade, the exact explanation of how the mutated pyrin gene, also known as MEFV (mediterranean fever), causes inflammation remains incomplete. The fact that pyrin is predominantly expressed in cells of myeloid origin such as neutrophils, monocytes and dendritic cells and that FMF has an apparent autosomal recessive pattern of inheritance, have led to the assumption that the inflammation in FMF is caused by inappropriate activation of the innate immune system and the result of reduced or complete loss of pyrin function. Pyrin has a complex structure and expression pattern. The full-length human pyrin, which has four domains, an N-terminal pyrin domain (PYD), followed by two B-box zinc-finger and coiled-coil domains and a C-terminal B30.2 domain, is expressed in the cytoplasm, whilst the short forms, which are created by caspase-1-mediated cleavage, migrate to the nucleus. Various overexpression experiments have demonstrated that pyrin can interact with apoptosis-associated speck-like protein containing a caspase recruitment domain (CARD) (ASC) to limit NLRP3 activation , but it can also bind capsase-1, via the B30.2 domain , to negatively regulate the enzyme activity. This latter effect of pyrin is particularly interesting as it provides a plausible explanation for how these mutations may affect pyrin function since most pathogenic mutations are in this B30.2 domain . However, overexpression of pyrin has also been shown to induce caspase-1 activation in cells that stably express ASC . Furthermore, short-forms of pyrin were shown to promote NF-κB activation and transcription of pro-inflammatory cytokines, the effects of which were particularly enhanced in cells harbouring the mutated protein . In addition, mice expressing truncated forms of pyrin were found not to have a phenotype resembling FMF , whilst the majority of pathogenic MEFV mutations are missense changes and, to date, no null mutations have been identified . All these observations suggest that pyrin mutations in FMF result in gain rather than loss of protein function. Further support for this notion comes from recent work that was done in pyrin-deficient and ‘knock-in (KI)’ mice harbouring mutant human B30.2 domains, since the murine pyrin homologue does not contain B30.2 domain . Similarly, to mice expressing the truncated protein , pyrin-deficient animals and heterozygous KI mice have no clinical features of FMF, suggesting that loss of pyrin function alone is insufficient to recreate the pro-inflammatory phenotype . On the other hand, mice with two mutated copies of the gene showed a number of inflammatory features consistent with the human disease, including generalised neutrophilia, dermatitis and arthritis, but the hemizygous animals showed no evidence of the disease . Taken together, these findings suggest that disease-associated mutations lead to gain of pyrin function but that disease expression depends on the amount of mutated protein produced. This might explain why certain heterozygous pyrin mutations result in disease manifestation, albeit of a milder phenotype . Furthermore, this might also explain why MEFV sequence changes have been identified in chronic inflammatory diseases, such as Behçet’s disease , systemic AA amyloidosis and CD, , where they are thought to have pro-inflammatory disease-modifying effects.
The role of dysregulated unfolded protein response in the complex pathophysiology of TRAPS
A variety of mechanisms have been suggested to explain how autosomal dominant mutations of TNFRSF1A lead to the clinical manifestations of TRAPS ( Fig. 2 ). Nevertheless, the link between these mutations and associated phenotypes remains poorly understood with much apparent clinical heterogeneity between different mutations as well as among patients with the same mutation.
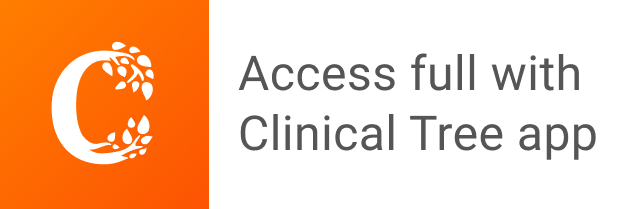