Understanding and recognizing artifacts and technical factors play a central role in every nerve conduction and electromyography (EMG) study ( Box 8–1 ). The value of the information gained during an electrodiagnostic (EDX) study relies on two important and complementary processes: (1) collecting the data correctly and (2) interpreting the data correctly. If the collected data are not technically accurate, then correct interpretation of the data can never occur, either at the time of the study or later by the referring physician.
Physiologic Factors
Temperature
Age
Height
Proximal versus distal nerve segments
Anomalous innervations (see Chapter 7 )
Non-physiologic Factors
Electrode impedance mismatch and 60 Hz interference
Filters
Electronic averaging
Stimulus artifact
Cathode position: reversing stimulator polarity
Supramaximal stimulation
Co-stimulation of adjacent nerves
Electrode placement for motor studies
Antidromic versus orthodromic recording
Distance between recording electrodes and nerve
Distance between active and reference recording electrodes
Limb position and distance measurements
Limb position and waveform morphology
Sweep speed and sensitivity
EDX studies rely upon acquiring and amplifying very small bioelectric signals in the microvolt and millivolt range. This process is technically demanding, because a large number of physiologic and non-physiologic factors can significantly interfere with the accuracy of the data. Physiologic factors, such as limb temperature and age, as well as non-physiologic factors, such as electrode impedance and electrical noise, are equally important. Failure to recognize these technical factors that influence the EDX study can result in type I errors: diagnosing an abnormality when none is present (i.e., convicting an innocent man), and type II errors: failing to recognize an abnormality when one is present (i.e., letting a guilty man go free). Although both are important, type I errors are potentially more serious. For example, “abnormalities” on EDX testing due to unrecognized technical errors can result in a patient being misdiagnosed with a condition they do not have. Such faulty diagnoses can lead to further inappropriate testing and treatment. Recognizing technical factors and other potential sources of error in the EDX laboratory is essential in improving the efficiency and validity of the EDX study and reducing patient discomfort.
Physiologic Factors
Temperature
Temperature is the most important of all the physiologic factors. It affects nearly every parameter measured in a nerve conduction study, including conduction velocity, distal latency, and waveform morphology. Temperature also can affect motor unit action potential (MUAP) morphology during the needle EMG examination. Physiologically, cooler temperatures result in delayed inactivation of sodium channels and subsequently prolong the time of depolarization (see Chapter 2 ). For myelinated fibers, conduction velocity is primarily determined by the time delay of depolarization that occurs at the nodes of Ranvier. Hence, prolonged depolarization times result in slowed conduction velocities for the nerve being studied. Conduction velocity slows in a fairly linear manner within the normal physiologic range of limb temperature (approximately 21–34°C). For motor and sensory conduction velocities, conduction velocity slows between 1.5 and 2.5 m/s for every 1°C drop in temperature, and distal latency prolongs by approximately 0.2 ms per degree.
In addition, longer channel opening time results in a larger influx of sodium. Subsequently, each nerve fiber depolarization is larger and longer. For both compound muscle action potentials (CMAPs) and sensory nerve action potentials (SNAPs), cooling results in a higher amplitude and longer duration as a consequence of larger and longer individual muscle and sensory fiber action potentials, respectively ( Figure 8–1 ). This effect is more pronounced in sensory fibers, because the duration of individual sensory nerve fiber action potentials normally is shorter than that of individual muscle fibers. The normal process of phase cancellation is more prominent when individual fiber action potential durations are shorter (see Chapter 3 ). Thus, when cooling occurs, individual sensory nerve fiber action potentials prolong, resulting in less phase cancellation and a higher compound nerve action potential. Accordingly, any sensory study that yields a high-amplitude, long-duration potential along with a slow conduction velocity should alert the electromyographer to a possible cooling effect.

Lowering the temperature has a similar though less marked effect on MUAPs measured during needle EMG. MUAP duration and amplitude increase with cooler temperatures; correspondingly, the number of phases also may increase.
There may be significant variation in limb temperature among individuals, even when ample time is allowed for temperature to equilibrate in a warm laboratory. Moreover, there is marked variation of temperature over the course of a given nerve, with a trend toward cooler temperatures as the nerve travels distally and superficially within the respective limb. Furthermore, in a warm limb, skin surface temperature typically is 1 to 2°C warmer compared to the near nerve temperature. The opposite often is true in a cool limb, where the skin surface temperature typically is cooler compared to the near nerve temperature.
It is easy to see how an electromyographer might mistakenly interpret a nerve conduction or needle EMG study as abnormal if a cool limb temperature is not appreciated and corrected. For example, a common mistake is to make a diagnosis of a polyneuropathy based on findings of slowed conduction velocities, prolonged distal latencies, and slightly large, polyphasic MUAPs that actually are due to cold limb temperatures. Another common misdiagnosis is that of a distal entrapment neuropathy. For example, prolonged distal median motor and sensory latencies in a cool limb may create the false impression of a median neuropathy at the wrist (i.e., carpal tunnel syndrome). Lastly, in patients with axonal peripheral neuropathies, cooling may slow nerve conduction velocity into the range associated with demyelination, which then could profoundly alter the electrodiagnostic impression and subsequent evaluation and treatment.
There are several ways to reduce the influence of cooling on the EDX study. First, the electromyographer must recognize the importance of temperature in every nerve conduction and EMG study ( Box 8–2 ). Distal limb temperatures should be routinely recorded and monitored in all patients and ideally maintained between 32 and 34°C. A normal temperature at the beginning of a study does not ensure that the limb will not cool down as the study progresses; in fact, it often does cool down.
Effects of Cool Temperature
Slowed nerve conduction velocity
Prolonged distal latency
Increased amplitude and duration of potentials on nerve conductions (SNAP > CMAP)
Increased duration, amplitude, and phases of MUAPs
Maintenance of Temperature
Measure distal limb temperature in all patients
Maintain temperature at 32–34°C with heating lamp, warm packs, or hydrocollator
Remember there may be a delay when heating between when the skin and the underlying nerve reach the desired temperature
If limb is profoundly cool (>10°C cooler than desired), immerse the limb in warm water and then maintain temperature with a heating lamp
If limb cannot be warmed, use conversion factors of 1.5–2.5 m/s/°C for conduction velocity and 0.2 m/s/°C for distal latency
CMAP, compound muscle action potential; MUAP, motor unit action potential; SNAP, sensory nerve action potential.
Limbs can be heated with heating lamps, warming packs or hydrocollators. The ideal way to warm and maintain proper limb temperature is to use a heating lamp device that has a feedback control mechanism from a temperature sensor placed on the distal hand or foot. Unfortunately, these devices are now very difficult to obtain because most manufacturers have stopped producing them due to concerns over litigation; there were incidents wherein patients were burned when they grabbed the heating element and did not realize it was a heating device. It is important to be aware, however, that regardless of the method used to warm the limb, there may be a significant delay between the time that the skin reaches the desired temperature and when the underlying nerve and muscle warm up. When a limb is warmed, skin temperature usually reaches the desired temperature several minutes before the underlying nerve and muscle do so. For limbs that are profoundly cool, it may require 20 to 40 minutes for the underlying nerve temperature to equilibrate ( Figure 8–2 ). If this fact is not recognized, the conduction velocity may increase through the EDX study as more time passes and the nerve warms up, despite a constant skin temperature. Nerves studied earlier in the examination will conduct more slowly than those studied later in the examination, after the underlying nerve has warmed up. This can result in confusing and difficult to interpret results.

If a limb is profoundly cool (e.g., more than 10°C cooler than desired), surface heating usually is inadequate or requires too much time to heat the limb adequately. In such situations, it is useful to immerse the limb in warm water and allow it to heat up over several minutes. Once the target temperature is reached, the proper temperature should be maintained; otherwise, the limb usually will cool down again during the course of the study.
The electromyographer must always keep in mind that a mildly to moderately slowed conduction velocity or a slightly to moderately prolonged latency could be the result of an initially cool limb or inadequate heating. If limb warming is not possible or is difficult to achieve (e.g., portable studies in the intensive care unit), then a correction factor should be used, most commonly 1.5 to 2.5 m/s/°C for conduction velocity and 0.2 m/s/°C for distal latency.
Several modern EMG machines can monitor limb temperature, and can be set to automatically report corrected values for conduction velocity and latency based on temperature. However, one should keep in mind that these correction factors are derived primarily from individuals with normal nerves, and as such they may not hold true for all diseased nerves . Hence, it always is preferable to warm or rewarm a limb than to use a correction factor . On needle EMG, there is no correction factor for MUAP duration, amplitude, or phases.
Age
Age most prominently affects nerve conduction velocity and waveform morphology at the extremes of age. One of the most important determinants of nerve conduction velocity is the presence and amount of myelin. The process of myelination is age dependent and begins in utero, with nerve conduction velocities in full-term infants approximately half those of adult normal values. Accordingly, nerve conduction velocities of 25 to 30 m/s are considered normal at birth but would be in the demyelinating range for an adult. Conduction velocity rapidly increases after birth, reaching approximately 75% of adult normal values by age 1 year and the adult range by age 3 to 5 years, when myelination is complete.
Conduction velocities decrease slightly with age in adults, most likely as a consequence of the normal loss of motor and sensory neurons that occur with aging. This is more prominent for individuals older than 60 years, in whom conduction velocity decreases approximately 0.5 to 4.0 m/s/decade. The effect is slightly more pronounced for sensory than for motor fibers.
For adults, one can use normal values based on age for nerve conduction velocities, which take into account these small age-related changes in conduction velocity. However, it is difficult to remember values from tables based on both the age range and the nerve being studied. More commonly, tables of normal control values provide a range of nerve conduction velocities, usually for subjects between ages 10 and 60, which take into account the normal variability within that age range. Generally, the lower limits of normal are provided. Additional correction factors of 0.5 to 4.0 m/s/decade can then be used for older patients. For example, a normal median motor conduction velocity in the adult population is 49 m/s. However, in a 90-year-old patient, a median motor conduction velocity of 46 m/s would be considered normal, within the expected range for advanced age.
Age also has an effect on CMAP and SNAP amplitudes. Again, most tables of normal control values provide a range of amplitudes that take into account normal variability for subjects between the ages of 10 and 60. Assessment of distal lower extremity sensory responses in an elderly individual, especially the commonly recorded sural sensory response, can be difficult. The sural sensory response in particular is often a small potential, and it may be hard to elicit in some older individuals. SNAP amplitudes are known to decrease substantially with advanced age. Some have estimated that the SNAP amplitude drops by up to 50% by age 70. Thus, very low amplitude or absent lower extremity sensory responses in patients of advanced age must always be interpreted with caution and not necessarily considered abnormal without other confirmatory data.
Age also affects many parameters on the needle EMG study. The most prominent effect is on MUAP duration. It is well known that as an individual ages, MUAP duration increases. In the years from birth through childhood, the duration increases due to the physiologic increase of muscle fiber and motor unit size as an individual grows. In later life, the normal aging process results in a slow dropout of motor units. Some normal reinnervation occurs to compensate, which results in slight prolongation of motor unit duration as the individual ages. For these reasons, it is important to compare MUAP durations on needle EMG with normal values based on age (see Chapter 15 ).
Height
Along with temperature and age, height also affects nerve conduction velocity. Taller individuals commonly have slower conduction velocities than do shorter individuals. This effect of nerve length also is reflected in the well-recognized finding that normal conduction velocities are slower in the lower extremities, where the limbs are longer, than in the upper extremities. For example, on average, the normal sural sensory conduction velocity typically is 5 m/s slower than the median sensory conduction velocity, and the peroneal and tibial motor velocities typically are 6 to 9 m/s slower than the median and ulnar motor conduction velocities.
Two separate factors likely account for the effect of height or limb length on conduction velocity. First, nerves taper as they proceed distally. In general, the taller the individual, the longer the limb and the more tapered the distal nerve. Because conduction velocity is directly proportional to nerve diameter, the more distally tapered nerves in taller individuals conduct more slowly. By the same reasoning, nerves in the leg conduct more slowly than those in the arm because of longer limb length and more distal tapering. Second, and not as well appreciated, is that limbs are cooler distally than proximally and the legs generally are cooler than the arms. Thus, conduction velocity slowing due to cooling usually is more prominent in the legs than in the arms.
Tables of normal nerve conduction values usually take into account the range of normal height. However, modifications must be made for individuals of extreme height, just as they are needed for extremes of age. In practice, the adjustment usually is no more than 2 to 4 m/s below the lower limit of normal. For example, for an individual who is 6 feet 10 inches tall, a tibial conduction velocity of 38 m/s (the normal lower limit of normal is 41 m/s) should be considered within the normal range because of the effect of height.
The effect of height is especially relevant to the interpretation of late responses (F responses and H reflexes). The circuitry of these responses extends twice the length of the limb for the F response and twice the length of the proximal lower limb for the H reflex. Normal values of absolute latency for these potentials must be based on limb length or height (see Chapter 4 ). Failure to do so will result in erroneously labeling of taller individuals as having “abnormal” late responses. In some situations, however, the effect of height is not relevant, as when latencies are compared between a symptomatic and a contralateral asymptomatic limb.
Proximal versus Distal Nerve Segments
Nerve conduction velocities vary between the distal and proximal segments of a limb, just as they vary with height, because of changes in nerve diameter and temperature. Proximal nerve segments tend to conduct slightly faster than distal segments in a normal individual. For example, the normal conduction velocity of the median nerve between the axilla and elbow is slightly faster than between the elbow and wrist. This is true for the same reasons that conduction velocity is faster in the arms than in the legs: (1) distal nerve segments are tapered and therefore conduct more slowly than proximal segments, and (2) distal limb segments are cooler than proximal segments and therefore conduct more slowly.
Nonphysiologic Factors
Electrode Impedance and Noise
Electrical noise is present in every EDX laboratory. The most common cause of electrical noise is 60 Hz interference generated by other electrical devices (e.g., lights, fans, heaters, computers). Outside of the EDX laboratory, particularly in the intensive care unit, there may be many other sources of electrical noise such as ventilators, monitors, and other electrical devices. This noise can create a host of problems, especially when recording very small potentials such as SNAPs or fibrillation potentials ( Figure 8–3 ). However, the examiner usually can reduce electrical noise to an acceptable level by paying close attention to technical details.

All signals recorded during the nerve conduction study and needle EMG are the result of differential amplification ( Figure 8–4 ). With differential amplification, the difference between the signals at the active (G1) and reference (G2) electrodes is amplified and then displayed. Thus, if the same electrical noise is present at both the active and reference electrodes, it will be subtracted out, and only the signal of interest will be amplified (this is known as common mode rejection).

The best way to achieve identical electrical noise at each electrode is to ensure that the impedance at each electrode is the same (i.e., to prevent electrode impedance mismatch). Impedance is an electrical term that combines the effects of resistance to flow for a direct current (DC), and capacitance and inductance for an alternating current (AC). One will recall from Ohm’s law that E = IR . The voltage ( E ), in this case the voltage from electrical noise, equals the current ( I ) induced from the electrical noise multiplied by the resistance ( R ), or impedance. If the resistance, or impedance, is different at the two electrodes, the same electrical noise will induce a different voltage at each electrode input. This difference will then be amplified and displayed, often obscuring the signal of interest.
The best way to eliminate 60 Hz interference is to ensure that each electrode appears identical to the amplifier ( Box 8–3 ). This can be accomplished by several steps. First, ensure that the electrodes are intact, without any frayed or broken connections. Next, the skin preparation should be thorough, using either alcohol or acetone to remove dirt and oil. Conducting electrode jelly is then applied to the electrode before it is attached to the skin. The recording electrodes should be held firmly against the skin with tape or a Velcro band. Finally, the closer the electrodes are to each other, the more likely any associated electrical noise will appear identical to a differential amplifier.
Active and reference recording electrodes should be the same type.
Ensure all contacts are intact without any frayed or broken connections.
Clean all dirt and oil from the skin using alcohol or acetone.
Apply conducting electrode jelly between the skin and electrodes.
Secure electrodes firmly to the skin with tape or Velcro straps.
Place ground between stimulator and recording electrodes.
Use coaxial recording cables.
Filters
Every potential recorded during nerve conduction studies and needle EMG passes through both a low- and a high-frequency filter before being displayed. The role of the filters is to faithfully reproduce the signal of interest while trying to exclude both low- and high-frequency electrical noise. Low-frequency (high-pass) filters exclude signals below a set frequency while allowing higher-frequency signals to pass through. High-frequency (low-pass) filters exclude signals above a certain frequency while allowing lower-frequency signals to pass through. Low-frequency noise (<10 Hz) results in wandering of the baseline (close to DC), whereas high-frequency noise (>10 kHz) commonly obscures high-frequency potentials (e.g., sensory nerve action potentials or fibrillation potentials).
By allowing the signal to pass through a certain “pass band,” some unwanted electrical noise can be excluded. The pass band varies for different EDX studies. For motor conduction studies, the low- and high-frequency filters typically are set at 10 and 10 kHz, respectively. For sensory conduction studies, the low- and high-frequency filters typically are set at 20 and 2 kHz, respectively. Note that the high-frequency filter is set lower for sensory than for motor nerve conduction studies. This is done in order to reduce high-frequency noise, which more easily interferes with the recording of sensory nerve action potentials, which contain higher-frequency components compared with motor potentials ( Figure 8–5 ).

The use of filters always involves some tradeoff. No filter, whether analog or digital, results in a sharp cutoff with complete exclusion of all signals above the high-frequency settings or below the low-frequency settings. It is essential to recognize that filtering also results in some loss or alteration of the signal of interest. For instance, as the low-frequency filter is reduced, more low-frequency signals pass through. This results in the duration of the recorded potential increasing slightly because the duration is primarily a lower frequency response. Likewise, as the high-frequency filter is lowered, more high-frequency signals are excluded. This results in the amplitude of the recorded potential usually decreasing because amplitude is primarily a higher frequency response ( Figure 8–6 ). Accordingly, all potentials should be obtained with standardized filter settings and should be compared only with normal values based on studies using the same filter settings.
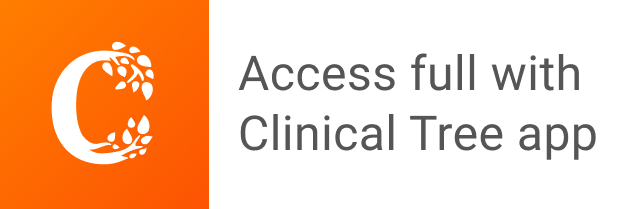