Research in tissue engineering has undoubtedly achieved significant milestones in recent years. Although it is being applied in several disciplines, tissue engineering’s application is particularly advanced in orthopedic surgery and in degenerative joint diseases. The literature is full of remarkable findings and trials using tissue engineering in articular cartilage disease. With the vast and expanding knowledge, and with the variety of techniques available at hand, the authors aimed to review the current concepts and advances in the use of cell sources in articular cartilage tissue engineering.
Key points
- •
The technology of tissue engineering has witnessed substantial advancements and innovations in the recent years and carries significant potential for treating cartilaginous disorders.
- •
Although a variety of techniques in articular cartilage are available, preliminary data for almost all are encouraging.
- •
Several cell sources exist for tissue engineering; however, many parameters need further optimization, including the best-suited use of each cell source.
- •
Tissue engineering is already impacting clinical practice and will surely play an essential role in the daily practice in the foreseeable future.
Introduction
Cartilage is a highly specialized connective tissue maintained by chondrocytes. Articular cartilage is a highly organized tissue that is prone to undergo many alterations resulting from aging, trauma, or inflammatory processes, the end result of which is tissue deterioration, functional impairment, and pain. Limited by the lack of vascularization and ability of migration of healthy chondrocytes to the damaged area, articular cartilage has a very limited ability to repair, making the management of the disease processes rather challenging. Currently, total hip and total knee replacement are the preferred surgical interventions for end-stage arthritis. More recently, tissue engineering and regenerative medicine have witnessed substantial advances, particularly with the introduction of new articular cartilage repair techniques.
Tissue engineering is a discipline aimed at restoring the functional role of various organs through the regeneration and formation of new tissues. It is being studied and applied in most organ systems, restoring the function of various tissues and organs, such as heart valves, blood vessels, the trachea, and bladder, among many others. In general, tissue-engineering application follows a general principle, albeit the presence of variances that occur within each step. The first step in tissue engineering is the proper choice of cells. After being harvested, cells are cultured in vitro by placing them into a biomaterial scaffold that allows for cell differentiation or proliferation. The system is then placed in a bioreactor, which provides the necessary signals for proper development. The tissue construct is finally implanted into the host ( Fig. 1 ). The aforementioned protocol simplification serves as a model, and it should be reiterated that multiple variations of this general protocol exist, such as cell-free techniques, among others. The purpose of this review is to present a current-concepts update on cell-based articular cartilage tissue engineering and briefly assess clinical trials using each cell source.

Chondrocyte-dependent Tissue Engineering
Various cell sources can be used in tissue engineering. A summary of the characteristics, main advantages, and disadvantages of each are presented in Tables 1 and 2 . Chondrocytes, being the cells responsible for cartilage synthesis, have long been studied and used in articular tissue engineering.
Cell Source | Advantages | Main Disadvantages and Challenges |
---|---|---|
Embryonic stem cells | Give rise to any cell type, unlimited proliferation | Scarce supply, ethical restrictions, immune compatibility |
iPS | Give rise to any cell type, avoid host/donor incompatibility, production of patient-specific cell types, no ethical restrictions | Avoiding accumulation of environmental DNA damage, avoiding genetic modifications when using viral integration, target cell purification, efficiency of reprogramming, time consumption and costs, optimizing cell source for reprogramming, incomplete overlap with ESCs |
MSCs | Give rise to a wide variety of cells, widely distributed and found, certain sources accessible with minimal invasive procedures, no ethical concerns, immune-compatible | Differentiation potential differs depending on origin, MSC lineage commitment dependent on various parameters to be resolved, phenotypic changes by prolonged passaging |
Direct conversion | Direct generation of cells from fibroblasts with no need to revert to pluripotency | Limited cell types can be produced, time consuming, require further studies for optimization |
Terminally differentiated cells | No need to induce differentiation, cells of interest already present | Scarce supply, limited proliferation capacity, cannot act as cell sources for tissues damaged by tumors |
Autologous chondrocyte implantation
Autologous chondrocyte implantation (ACI) was introduced in 1989 and was applied in the clinical setting in 1994 for treatment of deep cartilage defects of the knee. Since its introduction, various clinical trials have been conducted to assess the outcome and validity of the intervention. ACI is a 2-stage process:
- 1.
First step, harvest and growth: arthroscopic excision of a biopsy from a healthy non-load- or low-load-bearing articular cartilage.
- a.
Cartilage is then treated with enzymes in order to release chondrocytes.
- b.
Followed by in vitro expansion to up to 48 million cells.
- a.
- 2.
Second step, debridement and implantation: following the surgical debridement of the damaged cartilage, the chondrocytes are implanted into the injured area to healthy articular tissue and covered by a membrane, usually a periosteal flap or collagen sheet to void cell leakage.
Various studies and trials reported positive short- and long-term follow-up outcomes with ACI technique. However, with the current studies at hand, the best use of ACI is still not well formulated. More work is needed to define the settings where ACI provides the best functional and/or financial outcome compared with the current standard treatments. The challenges that exist for ACI include prevention of cell leakage from the repair site and a high reoperation rate.
Chondrocyte-seeded scaffolds and matrix-induced autologous chondrocyte implantation
Similar to ACI, matrix-induced autologous chondrocyte implantation (MACI) is a 2-step procedure involving donor-site chondrocyte extraction followed by implantation. However, MACI uses an extra step in which the expanded chondrocytes are seeded into a 3-dimensional (3D) matrix made of porcine-derived mixed collagen (type I and III). Chondrocyte-seeded scaffolds are numerous and include hyaluronan-based scaffolds, bioresorbable poly(lactic coglycolic acid) polymers, and agarose-alginate hydrogel, among many others. In general, the use of scaffold aims at replicating the in vivo conditions in which the cells survive. This approach allows for the following:
- 1.
Proper cellular growth and differentiation
- 2.
Maintenance of the chondrogenic phenotype of the cells
- 3.
Balanced and homogeneous distribution of chondrocytes and cartilage formation ensuring mechanical stability
The tissue construct is thus more robust and capable of mimicking the crucial characteristics of the articular cartilage. Although ACI requires cells to be injected, in scaffold-based techniques, the cell-based scaffold is sutured or glued to the damaged cartilage. Although MACI has shown beneficial outcomes, independent of the scaffold material used, the procedure has not yet been proven superior to ACI or other standard interventions. A recent systematic review asserted the need of more large-scale studies to dictate the optimal clinical application of MACI.
ACI’s most reported adverse effect is hypertrophy of the flap used in sealing the implanted cells. Other adverse effects include postoperative pain as well as scar formation and limited mobility and fibroarthrosis secondary to the arthrotomy. In addition, the need for a perifocal solid cartilage onto which the periosteal flap or collage sheet would be fixed narrows the applicability of ACI to focal cartilage lesions. In contrast, scaffold-based MACI allows a better filling of the defect, is less technically challenging, has greater graft stability resulting in shorter recovery period, and can be used in diffuse defects when grafts can be fixed directly into subchondral bone. Disadvantages of both techniques include cost, procedure complexity, and potential of chondrocyte dedifferentiation in culture into fibroblast-like cells.
More recently, tissue engineering has been applying more prolonged forms of 3D seeding. In MACI, chondrocytes are seeded for 3 days, after which the tissue is implanted. This seeding technique might constitute an insufficient time for tissue organization, extracellular matrix (ECM) deposition, and achievement of biochemical integrity, rendering the tissue fragile and incompetent. To that end, new methods are implementing longer culturing periods, extending up to 6 weeks. The longer duration permits ample time for chondrocytes to proliferate, mature, deposit their own ECM, and achieve functional standards of mechanical robustness. Aside from maintaining cells in their regular microenvironment of ECM, multiple confounding variables can impact the development and growth of cells, especially in terms of maintaining and inducing cellular differentiation. As chondrocytes expand in culture to achieve an adequate number of cells, the cells lose their chondrogenic phenotype with each passage. Minimizing dedifferentiation can be attained by decreasing the number of passages, optimizing expansion medium components (ie, growth factors), 3D culturing, and exogenous mechanical stress. The use of growth factors for chondrocyte expansion is an ongoing field of research. FGF-2 has been shown to maintain chondrogenic phenotype, induce proliferation, and promote neocartilage with higher matrix content. Many other factors have been reported to play a pivotal role in chondrocyte proliferation and differentiation, including EGF, TGF-B1, and BMPs, among others. Scaffolds used in cartilage tissue engineering are numerous and beyond the scope of this review. However, such scaffolds are generally divided into microporous (hydrogels) and macroporous scaffolds, each with its own advantages and disadvantages. Biomemetic stimuli are also known to play integral roles in chondrocyte differentiation and development. A main exogenous stimulus in 3D culture is mechanical stimulation. Cartilage endures mechanical stress and continuous load bearing, and thus, the designed neotissue must be able to mirror this capacity and withhold regular joint loads. Chondrocytes are known to proliferate and produce more ECM in response to mechanical loads, such as hydrostatic pressure and dynamic compression, among others. However, applying mechanical stimuli in a consistent manner and in large scale remains a technical challenge. Other relevant exogenous stimuli include hypoxia, which increases ECM deposition and improves the biochemical properties of collagen, pH, direct perfusion, the use of chemicals and enzymes, and coculturing with mesenchymal stem cells (MSCs).
Scaffold-free technologies rely on culturing chondrocytes at high densities. Over a couple of weeks, cells secrete ECM while adhering together, which results in forming the final robust neotissue. Stimulation of these constructs with exogenous stimuli has been shown to result in tissue with similar properties and qualities of normal in vivo cartilage. Clinical trials are being conducted on both Chondrosphere (Teltow, Germany) and RevaFle (St. Louis, MO, USA), the 2 main scaffold-free constructs.
Stem Cell-based Tissue Engineering
Chondrocyte-dependent cartilage regeneration remains costly and invasive and carries the risk of dedifferentiation into a fibroblast-like stage. Thus, stem cells have been explored as an alternative source of cells.
Mesenchymal stem cells
MSCs hold a true promise for tissue engineering. MSCs are capable of differentiating into bone, cartilage, adipose tissue, muscle, neural, endothelial, and insulin-producing cells. MSCs retain their multilineage differentiation potential in culture with an extensive proliferative ability in an uncommitted state. “Mesenchymal stem cells” is a terminology used to refer to stromal cells with specific properties. The International Society for Cellular Therapy proposed a standard set of rules to define the identity of these cells :
- 1.
MSCs should be plastic adherent, forming fibroblast-like colonies
- 2.
MSCs should be able to differentiate into various specialized cell lineages
- 3.
Ability to express defined cell surface marker profiles
Although there is a no definite set of markers, the agreed upon markers of MSCs include specific immunophenotypic marker combinations (CD73, CD90, and CD105) and lack both hematopoietic markers (CD11b, CD19, CD34, and CD45) and class II major histocompatibility complex (MHC) molecules (HLA-DR). MSCs have various sources such as bone marrow (BM-MSCs), muscle, liver, synovium, umbilical cord blood (UC-MSCs), placenta and amnion, peripheral blood, pancreas, dental tissue, adipose tissue (ASCs), and urine. The wide variety and large number of sources, capability of differentiating into various types of specific-cell lineages, minimal ethical concerns, and immune-compatibility make MSCs a robust viable option in tissue engineering and regenerative medicine.
Bone marrow mesenchymal stem cells
BM-MSCs are the most studied MSCs for cartilage tissue engineering. BM-MSCs from a diseased individual have been shown to produce cartilage similar to that of healthy MSCs donors and maintain the chondrogenic phenotype in the presence of TGf-b. As well, BM-MSCs have been shown to maintain an anti-inflammatory state in diseased organs. In general, these cells have been implemented in the clinical setting in 2 ways:
- 1.
Intra-articular injection of the cells
- 2.
Using an MACI-like approach
The beneficial outcomes of intra-articular injection have been reported in preclinical studies, case series, as well as randomized studies. The MACI-like technique has also shown promising results, albeit less evidence and support in the literature. Culturing BM-MSCs on scaffolds before implantation has shown good functional outcomes in several studies. However, some studies also noted no benefit of BM-MSC’s administration per se when used as an add-on to the current standard procedure of microfracture. Nevertheless, the beneficial effect stems from the minimal invasive technique used in the BM-MSC group.
In summary, the use of BM-MSCs has been showing substantial promise. Direct injection of BM-MSCs into diseased cartilage has shown beneficial outcomes comparable to ACI, with the added benefit of being less costly and avoiding the risk of an additional surgery and donor site morbidity. However, many parameters still need to be optimized, including dosing regimens, and results of current and further trials must be analyzed to ensure safety of MSCs, effectiveness over the long-term and comparative efficacy to other available treatments.
Umbilical cord derived mesenchymal stem cells
Umbilical cord blood has been shown to contain stem cells with mesenchymal properties capable of differentiating into various cell types including chondrocytes. Collection of umbilical cord derived MSCs (UC-MSCs) is noninvasive and relatively easy, with not much of an ethical debate generated. To date, no human clinical trial was conducted to assess the efficacy of cartilage generated using UC-MSCs. However, results of a limited number of preclinical studies seem encouraging. In one study, using UC-MSCs with a hyaluronic acid hydrogel composite in minipigs resulted in a superior and more complete hyaline cartilage regeneration than the control group. Another study using rat models also showed significant improvement in the knee receiving UC-MSCs compared with the contralateral one. Although UC-MSCs are showing positive results in preclinical trials, it is still too early to draw a conclusion, especially with the lack of human clinical trials.
Adipose tissue–derived mesenchymal stem cells
ASCs have been regarded as a desirable cell choice for various reasons:
- 1.
Readily accessible
- 2.
Availability in large quantities
- 3.
Capability of differentiation similar to BM-MSCs
- 4.
Lack of immunogenicity
- 5.
Anti-inflammatory properties
- 6.
Minimal ethical considerations
In a study of 18 patients, intra-articular injection of ASCs resulted in improved function and pain of the knee joint with documented regeneration of hyaline-like articular cartilage. The beneficial effects of ASCs on cartilage regeneration were also evident in 3 other trials, with a total of 133 patients. One of the studies found improved results of scaffold-based ASCs compared with ASCs injection alone. Other early studies have shown improvement in validated outcomes scores and pain scores but have not shown evidence of cartilage regeneration, one of the primary goals of stem cell technology. The feasibility and positive outcomes of ASCs injection were also documented in a study of an elderly population with cartilage healing, functional improvement, and pain reduction. Similar to BM-MSCs, although initial results are encouraging, more research and trials should be invested in this promising technology.
Peripheral blood–derived mesenchymal stem cells
Peripheral blood has been found to be a source of MSCs capable of differentiating into chondrocytes. Even though limited in number, the effect of PB-MSCs on cartilage repair has been largely positive in all clinical trials conducted so far. The earliest study was conducted in 2011 on 5 patients, showing the proof of concept of using PB-MSCs for cartilage repair. Following that, 4 more clinical trials were conducted, demonstrating beneficial effects with clinical improvement and histologic evidence of substantial deposition of chondrogenic ECM.
Synovial-derived mesenchymal stem cells
In vitro and preclinical studies had shown the differentiation capacity as well as the beneficial effect of synovial-derived MSCs on cartilage repair. Human trials however had been limited. In one study, clinical outcome was improved, and documented healing was observed on MRI and second-look arthroscopy. Interestingly, the second trial showed a significant improvement using synovial-derived MSCs compared with MACI. However, this trial had a relatively small sample size of only 14 participants.
Other mesenchymal stem cells
Other tissue sources have been reported to harbor cells with chondrogenic differentiation potential. These other tissue sources include dermal stem cells, muscle-derived MSCs, placenta-derived MSCs, ear elastic cartilage, and periosteum.
Induced Pluripotent Stem Cells
Induced pluripotent stem cells (iPS) are autologous, somatic cells that undergo nuclear reprogramming into a primordial, embryonic stem cell–like state. iPS cells have the hallmarks of ES cell, including the following:
- 1.
Morphology
- 2.
Unlimited self-renewal
- 3.
Expression of key pluripotency genes
- 4.
Normal karyotype
In addition, human iPS cells have been proven to undergo functional differentiation into specialized cell lineages of all 3 embryonic germ layers. Reprogramming aims to induce differentiated cells to reverting to pluripotency. Once pluripotency is achieved, differentiation into almost any cell type can be achieved. The initial description of generating iPS was from Yamanaka in which introducing only 4 transcription factors (Oct3/4, c-Myc, Sox2, and Klf4) reprogrammed mouse adult fibroblasts into a pluripotent state. Following that, a variety of cells were used to induce pluripotency using different methods, including hair follicle dermal papillae, umbilical vein endothelial cells, hepatocytes, melanocytes, adipose tissue, peripheral blood cell, and many others. iPS were shown to differentiate into cartilage in vitro and actually had proven beneficial effect in preclinical studies. Although promising, evidence supporting iPS is still in an early stage compared with other cell sources. Many issues need to be resolved, including the differentiation capacity and chondrogenic phenotype in the long run, the potential for teratogenesis, resolving any carry-over undesired “epigenetic memory” and further in vivo testing.
Direct lineage conversion
More recently, scientists were able to induce cell-to-cell differentiation directly, bypassing the need to induce a pluripotent state. Direct conversion currently uses fibroblasts as the cell source and has been shown to be able to produce macrophages, neuronal cells, hepatocytes, multilineage blood cells, and cardiomyocytes. Fibroblasts have also been directly converted into chondrocytes with documented chondrogenic ECM deposition. In one pioneering study implementing direct conversion using cell sources other than fibroblasts, placental-derived cells were used for direct conversion into chondrocytes. The advent of transdifferentiation is certainly a critical step in the advances of tissue engineering; however, further research and investigation are required before being able to take it from the bench side to rigorous in vivo studies.
Summary
This review focused on tissue engineering techniques for cartilage repair that uses cell sources. However, other cell-free techniques are already being applied for cartilage repair. The technology of tissue engineering has witnessed substantial advancements and innovations in recent years and carries significant potential for treating cartilaginous disorders. Although much work is still required to truly develop and mimic the native human cartilage with its various aspects, this goal is much closer to reality with each day.
Disclosures: No additional funding sources were used for this article.
Conflicts of Interest: No conflicts of interest are evident for authors of this article.
References
- 1. Vinatier C., and Guicheux J.: Cartilage tissue engineering: from biomaterials and stem cells to osteoarthritis treatments. Ann Phys Rehabil Med 2016; 59: pp. 139-144
- 2. Makris E.A., Gomoll A.H., Malizos K.N., et al: Repair and tissue engineering techniques for articular cartilage. Nat Rev Rheumatol 2015; 11: pp. 21-34
- 3. Jones D.G., and Peterson L.: Autologous chondrocyte implantation. J Bone Joint Surg Am 2006; 88: pp. 2502-2520
- 4. Cole B.J., Pascual-Garrido C., and Grumet R.C.: Surgical management of articular cartilage defects in the knee. J Bone Joint Surg Am 2009; 91: pp. 1778-1790
- 5. Mankin H.J.: The response of articular cartilage to mechanical injury. J Bone Joint Surg Am 1982; 64: pp. 460-466
- 6. Mahomed N.N., Barrett J.A., Katz J.N., et al: Rates and outcomes of primary and revision total hip replacement in the United States Medicare population. J Bone Joint Surg Am 2003; 85-A: pp. 27-32
- 7. Hawker G., Wright J., Coyte P., et al: Health-related quality of life after knee replacement. J Bone Joint Surg Am 1998; 80: pp. 163-173
- 8. Mandl L.A.: Determining who should be referred for total hip and knee replacements. Nat Rev Rheumatol 2013; 9: pp. 351-357
- 9. Kusuma S., and Gerecht S.: Engineering blood vessels using stem cells: innovative approaches to treat vascular disorders. Expert Rev Cardiovasc Ther 2010; 8: pp. 1433-1445
- 10. Siemionow M., Bozkurt M., and Zor F.: Regeneration and repair of peripheral nerves with different biomaterials: review. Microsurgery 2010; 30: pp. 574-588
- 11. Sun H., Liu W., Zhou G., et al: Tissue engineering of cartilage, tendon and bone. Front Med 2011; 5: pp. 61-69
- 12. Shin Y.S., Choi J.W., Park J.K., et al: Tissue-engineered tracheal reconstruction using mesenchymal stem cells seeded on a porcine cartilage powder scaffold. Ann Biomed Eng 2015; 43: pp. 1003-1013
- 13. Stocum D.L., and Zupanc G.K.: Stretching the limits: stem cells in regeneration science. Dev Dyn 2008; 237: pp. 3648-3671
- 14. Togel F., and Westenfelder C.: Adult bone marrow-derived stem cells for organ regeneration and repair. Dev Dyn 2007; 236: pp. 3321-3331
- 15. Sundelacruz S., and Kaplan D.L.: Stem cell- and scaffold-based tissue engineering approaches to osteochondral regenerative medicine. Semin Cell Dev Biol 2009; 20: pp. 646-655
- 16. Howard D., Buttery L.D., Shakesheff K.M., et al: Tissue engineering: strategies, stem cells and scaffolds. J Anat 2008; 213: pp. 66-72
- 17. Kusano T., Jakob R.P., Gautier E., et al: Treatment of isolated chondral and osteochondral defects in the knee by autologous matrix-induced chondrogenesis (AMIC). Knee Surg Sports Traumatol Arthrosc 2012; 20: pp. 2109-2115
- 18. Benthien J.P., and Behrens P.: The treatment of chondral and osteochondral defects of the knee with autologous matrix-induced chondrogenesis (AMIC): method description and recent developments. Knee Surg Sports Traumatol Arthrosc 2011; 19: pp. 1316-1319
- 19. Grande D.A., Pitman M.I., Peterson L., et al: The repair of experimentally produced defects in rabbit articular cartilage by autologous chondrocyte transplantation. J Orthop Res 1989; 7: pp. 208-218
- 20. Brittberg M., Lindahl A., Nilsson A., et al: Treatment of deep cartilage defects in the knee with autologous chondrocyte transplantation. N Engl J Med 1994; 331: pp. 889-895
- 21. Bartlett W., Skinner J.A., Gooding C.R., et al: Autologous chondrocyte implantation versus matrix-induced autologous chondrocyte implantation for osteochondral defects of the knee: a prospective, randomised study. J Bone Joint Surg Br 2005; 87: pp. 640-645
- 22. Ringe J., Burmester G.R., and Sittinger M.: Regenerative medicine in rheumatic disease-progress in tissue engineering. Nat Rev Rheumatol 2012; 8: pp. 493-498
- 23. Saris D.B., Vanlauwe J., Victor J., et al: Treatment of symptomatic cartilage defects of the knee: characterized chondrocyte implantation results in better clinical outcome at 36 months in a randomized trial compared to microfracture. Am J Sports Med 2009; 37: pp. 10S-19S
- 24. Saris D.B., Vanlauwe J., Victor J., et al: Characterized chondrocyte implantation results in better structural repair when treating symptomatic cartilage defects of the knee in a randomized controlled trial versus microfracture. Am J Sports Med 2008; 36: pp. 235-246
- 25. Peterson L., Vasiliadis H.S., Brittberg M., et al: Autologous chondrocyte implantation: a long-term follow-up. Am J Sports Med 2010; 38: pp. 1117-1124
- 26. Minas T., Von Keudell A., Bryant T., et al: The John Insall Award: a minimum 10-year outcome study of autologous chondrocyte implantation. Clin Orthop Relat Res 2014; 472: pp. 41-51
- 27. Behery O.A., Harris J.D., Karnes J.M., et al: Factors influencing the outcome of autologous chondrocyte implantation: a systematic review. J Knee Surg 2013; 26: pp. 203-211
- 28. Bentley G., Biant L.C., Vijayan S., et al: Minimum ten-year results of a prospective randomised study of autologous chondrocyte implantation versus mosaicplasty for symptomatic articular cartilage lesions of the knee. J Bone Joint Surg Br 2012; 94: pp. 504-509
- 29. Van Assche D., Staes F., Van Caspel D., et al: Autologous chondrocyte implantation versus microfracture for knee cartilage injury: a prospective randomized trial, with 2-year follow-up. Knee Surg Sports Traumatol Arthrosc 2010; 18: pp. 486-495
- 30. Knutsen G., Drogset J.O., Engebretsen L., et al: A randomized trial comparing autologous chondrocyte implantation with microfracture. Findings at five years. J Bone Joint Surg Am 2007; 89: pp. 2105-2112
- 31. Behrens P., Bitter T., Kurz B., et al: Matrix-associated autologous chondrocyte transplantation/implantation (MACT/MACI)–5-year follow-up. Knee 2006; 13: pp. 194-202
- 32. Nehrer S., Domayer S., Dorotka R., et al: Three-year clinical outcome after chondrocyte transplantation using a hyaluronan matrix for cartilage repair. Eur J Radiol 2006; 57: pp. 3-8
- 33. Sha’ban M., Kim S.H., Idrus R.B., et al: Fibrin and poly(lactic-co-glycolic acid) hybrid scaffold promotes early chondrogenesis of articular chondrocytes: an in vitro study. J Orthop Surg Res 2008; 3: pp. 17
- 34. Selmi T.A., Verdonk P., Chambat P., et al: Autologous chondrocyte implantation in a novel alginate-agarose hydrogel: outcome at two years. J Bone Joint Surg Br 2008; 90: pp. 597-604
- 35. Huang B.J., Hu J.C., and Athanasiou K.A.: Cell-based tissue engineering strategies used in the clinical repair of articular cartilage. Biomaterials 2016; 98: pp. 1-22
- 36. Sittinger M., and Burmester G.R.: Can engineered cartilage transplants be used for treating rheumatic diseases? Nat Clin Pract Rheumatol 2006; 2: pp. 172-173
- 37. Ringe J., and Sittinger M.: Tissue engineering in the rheumatic diseases. Arthritis Res Ther 2009; 11: pp. 211
- 38. Moutos F.T., and Guilak F.: Composite scaffolds for cartilage tissue engineering. Biorheology 2008; 45: pp. 501-512
- 39. Zhang L., Hu J., and Athanasiou K.A.: The role of tissue engineering in articular cartilage repair and regeneration. Crit Rev Biomed Eng 2009; 37: pp. 1-57
- 40. Kon E., Di Martino A., Filardo G., et al: Second-generation autologous chondrocyte transplantation: MRI findings and clinical correlations at a minimum 5-year follow-up. Eur J Radiol 2011; 79: pp. 382-388
- 41. Ossendorf C., Kaps C., Kreuz P.C., et al: Treatment of posttraumatic and focal osteoarthritic cartilage defects of the knee with autologous polymer-based three-dimensional chondrocyte grafts: 2-year clinical results. Arthritis Res Ther 2007; 9: pp. R41
- 42. Marlovits S., Aldrian S., Wondrasch B., et al: Clinical and radiological outcomes 5 years after matrix-induced autologous chondrocyte implantation in patients with symptomatic, traumatic chondral defects. Am J Sports Med 2012; 40: pp. 2273-2280
- 43. Zheng M.H., Willers C., Kirilak L., et al: Matrix-induced autologous chondrocyte implantation (MACI): biological and histological assessment. Tissue Eng 2007; 13: pp. 737-746
- 44. Zeifang F., Oberle D., Nierhoff C., et al: Autologous chondrocyte implantation using the original periosteum-cover technique versus matrix-associated autologous chondrocyte implantation: a randomized clinical trial. Am J Sports Med 2010; 38: pp. 924-933
- 45. Ebert J.R., Robertson W.B., Woodhouse J., et al: Clinical and magnetic resonance imaging-based outcomes to 5 years after matrix-induced autologous chondrocyte implantation to address articular cartilage defects in the knee. Am J Sports Med 2011; 39: pp. 753-763
- 46. Kon E., Filardo G., Di Matteo B., et al: Matrix assisted autologous chondrocyte transplantation for cartilage treatment: a systematic review. Bone JointRes 2013; 2: pp. 18-25
- 47. Dehne T., Karlsson C., Ringe J., et al: Chondrogenic differentiation potential of osteoarthritic chondrocytes and their possible use in matrix-associated autologous chondrocyte transplantation. Arthritis Res Ther 2009; 11: pp. R133
- 48. Smeriglio P., Lai J.H., Yang F., et al: 3D hydrogel scaffolds for articular chondrocyte culture and cartilage generation. J Vis Exp 2015; 104: pp. 1-6
- 49. Caron M.M., Emans P.J., Coolsen M.M., et al: Redifferentiation of dedifferentiated human articular chondrocytes: comparison of 2D and 3D cultures. Osteoarthritis Cartilage 2012; 20: pp. 1170-1178
- 50. Mandl E.W., van der Veen S.W., Verhaar J.A., et al: Serum-free medium supplemented with high-concentration FGF2 for cell expansion culture of human ear chondrocytes promotes redifferentiation capacity. Tissue Eng 2002; 8: pp. 573-580
- 51. Mandl E.W., van der Veen S.W., Verhaar J.A., et al: Multiplication of human chondrocytes with low seeding densities accelerates cell yield without losing redifferentiation capacity. Tissue Eng 2004; 10: pp. 109-118
- 52. Jeon J.E., Schrobback K., Hutmacher D.W., et al: Dynamic compression improves biosynthesis of human zonal chondrocytes from osteoarthritis patients. Osteoarthritis Cartilage 2012; 20: pp. 906-915
- 53. Mandl E.W., Jahr H., Koevoet J.L., et al: Fibroblast growth factor-2 in serum-free medium is a potent mitogen and reduces dedifferentiation of human ear chondrocytes in monolayer culture. Matrix Biol 2004; 23: pp. 231-241
- 54. Martin I., Suetterlin R., Baschong W., et al: Enhanced cartilage tissue engineering by sequential exposure of chondrocytes to FGF-2 during 2D expansion and BMP-2 during 3D cultivation. J Cell Biochem 2001; 83: pp. 121-128
- 55. Yang K.G., Saris D.B., Geuze R.E., et al: Impact of expansion and redifferentiation conditions on chondrogenic capacity of cultured chondrocytes. Tissue Eng 2006; 12: pp. 2435-2447
- 56. Vivien D., Galera P., Lebrun E., et al: Differential effects of transforming growth factor-beta and epidermal growth factor on the cell cycle of cultured rabbit articular chondrocytes. J Cell Physiol 1990; 143: pp. 534-545
- 57. Shepard J.B., Jeong J.W., Maihle N.J., et al: Transient anabolic effects accompany epidermal growth factor receptor signal activation in articular cartilage in vivo. Arthritis Res Ther 2013; 15: pp. R60
- 58. van der Kraan P., Vitters E., and van den Berg W.: Differential effect of transforming growth factor beta on freshly isolated and cultured articular chondrocytes. J Rheumatol 1992; 19: pp. 140-145
- 59. Hwang N.S., Kim M.S., Sampattavanich S., et al: Effects of three-dimensional culture and growth factors on the chondrogenic differentiation of murine embryonic stem cells. Stem Cells 2006; 24: pp. 284-291
- 60. Elder B.D., and Athanasiou K.A.: Systematic assessment of growth factor treatment on biochemical and biomechanical properties of engineered articular cartilage constructs. Osteoarthritis Cartilage 2009; 17: pp. 114-123
- 61. Appelman T.P., Mizrahi J., Elisseeff J.H., et al: The influence of biological motifs and dynamic mechanical stimulation in hydrogel scaffold systems on the phenotype of chondrocytes. Biomaterials 2011; 32: pp. 1508-1516
- 62. Mizuno S., Tateishi T., Ushida T., et al: Hydrostatic fluid pressure enhances matrix synthesis and accumulation by bovine chondrocytes in three-dimensional culture. J Cell Physiol 2002; 193: pp. 319-327
- 63. Smith R.L., Rusk S.F., Ellison B.E., et al: In vitro stimulation of articular chondrocyte mRNA and extracellular matrix synthesis by hydrostatic pressure. J Orthop Res 1996; 14: pp. 53-60
- 64. Hasanova G.I., Noriega S.E., Mamedov T.G., et al: The effect of ultrasound stimulation on the gene and protein expression of chondrocytes seeded in chitosan scaffolds. J Tissue Eng Regen Med 2011; 5: pp. 815-822
- 65. Xu X., Urban J.P., Tirlapur U.K., et al: Osmolarity effects on bovine articular chondrocytes during three-dimensional culture in alginate beads. Osteoarthritis Cartilage 2010; 18: pp. 433-439
- 66. Meretoja V.V., Dahlin R.L., Wright S., et al: The effect of hypoxia on the chondrogenic differentiation of co-cultured articular chondrocytes and mesenchymal stem cells in scaffolds. Biomaterials 2013; 34: pp. 4266-4273
- 67. Makris E.A., Hu J.C., and Athanasiou K.A.: Hypoxia-induced collagen crosslinking as a mechanism for enhancing mechanical properties of engineered articular cartilage. Osteoarthritis Cartilage 2013; 21: pp. 634-641
- 68. Das R.H., van Osch G.J., Kreukniet M., et al: Effects of individual control of pH and hypoxia in chondrocyte culture. J Orthop Res 2010; 28: pp. 537-545
- 69. Gharravi A.M., Orazizadeh M., and Hashemitabar M.: Direct expansion of chondrocytes in a dynamic three-dimensional culture system: overcoming dedifferentiation effects in monolayer culture. Artif Organs 2014; 38: pp. 1053-1058
- 70. Makris E.A., MacBarb R.F., Paschos N.K., et al: Combined use of chondroitinase-ABC, TGF-beta1, and collagen crosslinking agent lysyl oxidase to engineer functional neotissues for fibrocartilage repair. Biomaterials 2014; 35: pp. 6787-6796
- 71. Meretoja V.V., Dahlin R.L., Kasper F.K., et al: Enhanced chondrogenesis in co-cultures with articular chondrocytes and mesenchymal stem cells. Biomaterials 2012; 33: pp. 6362-6369
- 72. Meretoja V.V., Dahlin R.L., Wright S., et al: Articular chondrocyte redifferentiation in 3D co-cultures with mesenchymal stem cells. Tissue Eng C Methods 2014; 20: pp. 514-523
- 73. Athanasiou K.A., Eswaramoorthy R., Hadidi P., et al: Self-organization and the self-assembling process in tissue engineering. Annu Rev Biomed Eng 2013; 15: pp. 115-136
- 74. DuRaine G.D., Brown W.E., Hu J.C., et al: Emergence of scaffold-free approaches for tissue engineering musculoskeletal cartilages. Ann Biomed Eng 2015; 43: pp. 543-554
- 75. Gunja N.J., Uthamanthil R.K., and Athanasiou K.A.: Effects of TGF-beta1 and hydrostatic pressure on meniscus cell-seeded scaffolds. Biomaterials 2009; 30: pp. 565-573
- 76. Griffin M., Iqbal S.A., and Bayat A.: Exploring the application of mesenchymal stem cells in bone repair and regeneration. J Bone Joint Surg Br 2011; 93: pp. 427-434
- 77. Pittenger M.F., Mackay A.M., Beck S.C., et al: Multilineage potential of adult human mesenchymal stem cells. Science 1999; 284: pp. 143-147
- 78. Kim E.Y., Lee K.B., Yu J., et al: Neuronal cell differentiation of mesenchymal stem cells originating from canine amniotic fluid. Hum Cell 2014; 27: pp. 51-58
- 79. Manochantr S., Tantrawatpan C., Kheolamai P., et al: Isolation, characterization and neural differentiation potential of amnion derived mesenchymal stem cells. J Med Assoc Thai 2010; 93: pp. S183-S191
- 80. Oswald J., Boxberger S., Jorgensen B., et al: Mesenchymal stem cells can be differentiated into endothelial cells in vitro. Stem Cells 2004; 22: pp. 377-384
- 81. Augello A., Kurth T.B., and De Bari C.: Mesenchymal stem cells: a perspective from in vitro cultures to in vivo migration and niches. Eur Cell Mater 2010; 20: pp. 121-133
- 82. Horwitz E.M., Le Blanc K., Dominici M., et al: Clarification of the nomenclature for MSC: the International Society for Cellular Therapy position statement. Cytotherapy 2005; 7: pp. 393-395
- 83. Seeberger K.L., Eshpeter A., and Korbutt G.S.: Isolation and culture of human multipotent stromal cells from the pancreas. Methods Mol Biol 2011; 698: pp. 123-140
- 84. Zhang D., Wei G., Li P., et al: Urine-derived stem cells: a novel and versatile progenitor source for cell-based therapy and regenerative medicine. Genes Dis 2014; 1: pp. 8-17
- 85. Estrela C., Alencar A.H., Kitten G.T., et al: Mesenchymal stem cells in the dental tissues: perspectives for tissue regeneration. Braz Dent J 2011; 22: pp. 91-98
- 86. Mihu C.M., Mihu D., Costin N., et al: Isolation and characterization of stem cells from the placenta and the umbilical cord. Rom J Morphol Embryol 2008; 49: pp. 441-446
- 87. Tsagias N., Koliakos I., Karagiannis V., et al: Isolation of mesenchymal stem cells using the total length of umbilical cord for transplantation purposes. Transfus Med 2011; 21: pp. 253-261
- 88. Hass R., Kasper C., Bohm S., et al: Different populations and sources of human mesenchymal stem cells (MSC): a comparison of adult and neonatal tissue-derived MSC. Cell Commun Signal 2011; 9: pp. 12
- 89. Kafienah W., Mistry S., Dickinson S.C., et al: Three-dimensional cartilage tissue engineering using adult stem cells from osteoarthritis patients. Arthritis Rheum 2007; 56: pp. 177-187
- 90. Zhang Y., Pizzute T., and Pei M.: Anti-inflammatory strategies in cartilage repair. Tissue Eng B Rev 2014; 20: pp. 655-668
- 91. Vangsness C.T., Farr J., Boyd J., et al: Adult human mesenchymal stem cells delivered via intra-articular injection to the knee following partial medial meniscectomy: a randomized, double-blind, controlled study. J Bone Joint Surg Am 2014; 96: pp. 90-98
- 92. Emadedin M., Aghdami N., Taghiyar L., et al: Intra-articular injection of autologous mesenchymal stem cells in six patients with knee osteoarthritis. Arch Iran Med 2012; 15: pp. 422-428
- 93. Centeno C.J., Busse D., Kisiday J., et al: Increased knee cartilage volume in degenerative joint disease using percutaneously implanted, autologous mesenchymal stem cells. Pain Physician 2008; 11: pp. 343-353
- 94. Nejadnik H., Hui J.H., Feng Choong E.P., et al: Autologous bone marrow-derived mesenchymal stem cells versus autologous chondrocyte implantation: an observational cohort study. Am J Sports Med 2010; 38: pp. 1110-1116
- 95. Davatchi F., Abdollahi B.S., Mohyeddin M., et al: Mesenchymal stem cell therapy for knee osteoarthritis. Preliminary report of four patients. Int J Rheum Dis 2011; 14: pp. 211-215
- 96. Murphy J.M., Fink D.J., Hunziker E.B., et al: Stem cell therapy in a caprine model of osteoarthritis. Arthritis Rheum 2003; 48: pp. 3464-3474
- 97. Wong K.L., Lee K.B., Tai B.C., et al: Injectable cultured bone marrow-derived mesenchymal stem cells in varus knees with cartilage defects undergoing high tibial osteotomy: a prospective, randomized controlled clinical trial with 2 years’ follow-up. Arthroscopy 2013; 29: pp. 2020-2028
- 98. Kasemkijwattana C., Hongeng S., Kesprayura S., et al: Autologous bone marrow mesenchymal stem cells implantation for cartilage defects: two cases report. J Med Assoc Thai 2011; 94: pp. 395-400
- 99. Wakitani S., Imoto K., Yamamoto T., et al: Human autologous culture expanded bone marrow mesenchymal cell transplantation for repair of cartilage defects in osteoarthritic knees. Osteoarthritis Cartilage 2002; 10: pp. 199-206
- 100. Wakitani S., Mitsuoka T., Nakamura N., et al: Autologous bone marrow stromal cell transplantation for repair of full-thickness articular cartilage defects in human patellae: two case reports. Cell Transplant 2004; 13: pp. 595-600
- 101. Kuroda R., Ishida K., Matsumoto T., et al: Treatment of a full-thickness articular cartilage defect in the femoral condyle of an athlete with autologous bone-marrow stromal cells. Osteoarthritis Cartilage 2007; 15: pp. 226-231
- 102. Haleem A.M., Singergy A.A., Sabry D., et al: The clinical use of human culture-expanded autologous bone marrow mesenchymal stem cells transplanted on platelet-rich fibrin glue in the treatment of articular cartilage defects: a pilot study and preliminary results. Cartilage 2010; 1: pp. 253-261
- 103. Lee K.B., Wang V.T., Chan Y.H., et al: A novel, minimally-invasive technique of cartilage repair in the human knee using arthroscopic microfracture and injections of mesenchymal stem cells and hyaluronic acid–a prospective comparative study on safety and short-term efficacy. Ann Acad Med Singapore 2012; 41: pp. 511-517
- 104. Ishige I., Nagamura-Inoue T., Honda M.J., et al: Comparison of mesenchymal stem cells derived from arterial, venous, and Wharton’s jelly explants of human umbilical cord. Int J Hematol 2009; 90: pp. 261-269
- 105. McElreavey K.D., Irvine A.I., Ennis K.T., et al: Isolation, culture and characterisation of fibroblast-like cells derived from the Wharton’s jelly portion of human umbilical cord. Biochem Soc Trans 1991; 19: pp. 29S
- 106. Jeong S.Y., Kim D.H., Ha J., et al: Thrombospondin-2 secreted by human umbilical cord blood-derived mesenchymal stem cells promotes chondrogenic differentiation. Stem Cells 2013; 31: pp. 2136-2148
- 107. Zhang X., Hirai M., Cantero S., et al: Isolation and characterization of mesenchymal stem cells from human umbilical cord blood: reevaluation of critical factors for successful isolation and high ability to proliferate and differentiate to chondrocytes as compared to mesenchymal stem cells from bone marrow and adipose tissue. J Cell Biochem 2011; 112: pp. 1206-1218
- 108. Nagamura-Inoue T., and He H.: Umbilical cord-derived mesenchymal stem cells: their advantages and potential clinical utility. World J Stem Cells 2014; 6: pp. 195-202
- 109. Ha C.W., Park Y.B., Chung J.Y., et al: Cartilage repair using composites of human umbilical cord blood-derived mesenchymal stem cells and hyaluronic acid hydrogel in a minipig model. Stem Cells Transl Med 2015; 4: pp. 1044-1051
- 110. Park Y.B., Song M., Lee C.H., et al: Cartilage repair by human umbilical cord blood-derived mesenchymal stem cells with different hydrogels in a rat model. J Orthop Res 2015; 33: pp. 1580-1586
- 111. Manferdini C., Maumus M., Gabusi E., et al: Adipose-derived mesenchymal stem cells exert antiinflammatory effects on chondrocytes and synoviocytes from osteoarthritis patients through prostaglandin E2. Arthritis Rheum 2013; 65: pp. 1271-1281
- 112. Wang Y.H., Wu J.Y., Chou P.J., et al: Characterization and evaluation of the differentiation ability of human adipose-derived stem cells growing in scaffold-free suspension culture. Cytotherapy 2014; 16: pp. 485-495
- 113. Mehlhorn A.T., Niemeyer P., Kaschte K., et al: Differential effects of BMP-2 and TGF-beta1 on chondrogenic differentiation of adipose derived stem cells. Cell Prolif 2007; 40: pp. 809-823
- 114. Jo C.H., Lee Y.G., Shin W.H., et al: Intra-articular injection of mesenchymal stem cells for the treatment of osteoarthritis of the knee: a proof-of-concept clinical trial. Stem Cells 2014; 32: pp. 1254-1266
- 115. Kim Y.S., Choi Y.J., Suh D.S., et al: Mesenchymal stem cell implantation in osteoarthritic knees: is fibrin glue effective as a scaffold? Am J Sports Med 2015; 43: pp. 176-185
- 116. Koh Y.G., Kwon O.R., Kim Y.S., et al: Comparative outcomes of open-wedge high tibial osteotomy with platelet-rich plasma alone or in combination with mesenchymal stem cell treatment: a prospective study. Arthroscopy 2014; 30: pp. 1453-1460
- 117. Koh Y.G., Choi Y.J., Kwon O.R., et al: Second-look arthroscopic evaluation of cartilage lesions after mesenchymal stem cell implantation in osteoarthritic knees. Am J Sports Med 2014; 42: pp. 1628-1637
- 118. Koh Y.G., Choi Y.J., Kwon S.K., et al: Clinical results and second-look arthroscopic findings after treatment with adipose-derived stem cells for knee osteoarthritis. Knee Surg Sports Traumatol Arthrosc 2015; 23: pp. 1308-1316
- 119. Chong P.P., Selvaratnam L., Abbas A.A., et al: Human peripheral blood derived mesenchymal stem cells demonstrate similar characteristics and chondrogenic differentiation potential to bone marrow derived mesenchymal stem cells. J Orthop Res 2012; 30: pp. 634-642
- 120. Saw K.Y., Anz A., Merican S., et al: Articular cartilage regeneration with autologous peripheral blood progenitor cells and hyaluronic acid after arthroscopic subchondral drilling: a report of 5 cases with histology. Arthroscopy 2011; 27: pp. 493-506
- 121. Saw K.Y., Anz A., Jee C.S., et al: High tibial osteotomy in combination with chondrogenesis after stem cell therapy: a histologic report of 8 cases. Arthroscopy 2015; 31: pp. 1909-1920
- 122. Fu W.L., Ao Y.F., Ke X.Y., et al: Repair of large full-thickness cartilage defect by activating endogenous peripheral blood stem cells and autologous periosteum flap transplantation combined with patellofemoral realignment. Knee 2014; 21: pp. 609-612
- 123. Turajane T., Chaweewannakorn U., Larbpaiboonpong V., et al: Combination of intra-articular autologous activated peripheral blood stem cells with growth factor addition/preservation and hyaluronic acid in conjunction with arthroscopic microdrilling mesenchymal cell stimulation Improves quality of life and regenerates articular cartilage in early osteoarthritic knee disease. J Med Assoc Thai 2013; 96: pp. 580-588
- 124. Saw K.Y., Anz A., Siew-Yoke Jee C., et al: Articular cartilage regeneration with autologous peripheral blood stem cells versus hyaluronic acid: a randomized controlled trial. Arthroscopy 2013; 29: pp. 684-694
- 125. Orth P., Rey-Rico A., Venkatesan J.K., et al: Current perspectives in stem cell research for knee cartilage repair. Stem Cells Cloning 2014; 7: pp. 1-17
- 126. Hatsushika D., Muneta T., Nakamura T., et al: Repetitive allogeneic intraarticular injections of synovial mesenchymal stem cells promote meniscus regeneration in a porcine massive meniscus defect model. Osteoarthritis Cartilage 2014; 22: pp. 941-950
- 127. Horie M., Sekiya I., Muneta T., et al: Intra-articular injected synovial stem cells differentiate into meniscal cells directly and promote meniscal regeneration without mobilization to distant organs in rat massive meniscal defect. Stem Cells 2009; 27: pp. 878-887
- 128. Mak J., Jablonski C.L., Leonard C.A., et al: Intra-articular injection of synovial mesenchymal stem cells improves cartilage repair in a mouse injury model. Sci Rep 2016; 6: pp. 23076
- 129. Sekiya I., Muneta T., Horie M., et al: Arthroscopic transplantation of synovial stem cells improves clinical outcomes in knees with cartilage defects. Clin Orthop Relat Res 2015; 473: pp. 2316-2326
- 130. Akgun I., Unlu M.C., Erdal O.A., et al: Matrix-induced autologous mesenchymal stem cell implantation versus matrix-induced autologous chondrocyte implantation in the treatment of chondral defects of the knee: a 2-year randomized study. Arch Orthop Trauma Surg 2015; 135: pp. 251-263
- 131. Yoshiya S., and Dhawan A.: Cartilage repair techniques in the knee: stem cell therapies. Curr Rev Musculoskelet Med 2015; 8: pp. 457-466
- 132. Sanchez-Adams J., and Athanasiou K.A.: Dermis isolated adult stem cells for cartilage tissue engineering. Biomaterials 2012; 33: pp. 109-119
- 133. Kalpakci K.N., Brown W.E., Hu J.C., et al: Cartilage tissue engineering using dermis isolated adult stem cells: the use of hypoxia during expansion versus chondrogenic differentiation. PLoS One 2014; 9: pp. e98570
- 134. Kuroda R., Usas A., Kubo S., et al: Cartilage repair using bone morphogenetic protein 4 and muscle-derived stem cells. Arthritis Rheum 2006; 54: pp. 433-442
- 135. Hsu S.H., Huang T.B., Cheng S.J., et al: Chondrogenesis from human placenta-derived mesenchymal stem cells in three-dimensional scaffolds for cartilage tissue engineering. Tissue Eng Part A 2011; 17: pp. 1549-1560
- 136. Mizuno M., Kobayashi S., Takebe T., et al: Brief report: reconstruction of joint hyaline cartilage by autologous progenitor cells derived from ear elastic cartilage. Stem Cells 2014; 32: pp. 816-821
- 137. Li Q., Tang J., Wang R., et al: Comparing the chondrogenic potential in vivo of autogeneic mesenchymal stem cells derived from different tissues. Artif Cells Blood Substit Immobil Biotechnol 2011; 39: pp. 31-38
- 138. Hui J.H., Li L., Teo Y.H., et al: Comparative study of the ability of mesenchymal stem cells derived from bone marrow, periosteum, and adipose tissue in treatment of partial growth arrest in rabbit. Tissue Eng 2005; 11: pp. 904-912
- 139. Nelson T.J., Martinez-Fernandez A., and Terzic A.: Induced pluripotent stem cells: developmental biology to regenerative medicine. Nat Rev Cardiol 2010; 7: pp. 700-710
- 140. Jopling C., Boue S., and Izpisua Belmonte J.C.: Dedifferentiation, transdifferentiation and reprogramming: three routes to regeneration. Nat Rev Mol Cell Biol 2011; 12: pp. 79-89
- 141. Takahashi K., and Yamanaka S.: Induction of pluripotent stem cells from mouse embryonic and adult fibroblast cultures by defined factors. Cell 2006; 126: pp. 663-676
- 142. Koyanagi-Aoi M., Ohnuki M., Takahashi K., et al: Differentiation-defective phenotypes revealed by large-scale analyses of human pluripotent stem cells. Proc Natl Acad Sci U S A 2013; 110: pp. 20569-20574
- 143. Takahashi K., Okita K., Nakagawa M., et al: Induction of pluripotent stem cells from fibroblast cultures. Nat Protoc 2007; 2: pp. 3081-3089
- 144. Tsai S.Y., Bouwman B.A., Ang Y.S., et al: Single transcription factor reprogramming of hair follicle dermal papilla cells to induced pluripotent stem cells. Stem Cells 2011; 29: pp. 964-971
- 145. Panopoulos A.D., Ruiz S., Yi F., et al: Rapid and highly efficient generation of induced pluripotent stem cells from human umbilical vein endothelial cells. PLoS One 2011; 6: pp. e19743
- 146. Aoi T., Yae K., Nakagawa M., et al: Generation of pluripotent stem cells from adult mouse liver and stomach cells. Science 2008; 321: pp. 699-702
- 147. Utikal J., Maherali N., Kulalert W., et al: Sox2 is dispensable for the reprogramming of melanocytes and melanoma cells into induced pluripotent stem cells. J Cell Sci 2009; 122: pp. 3502-3510
- 148. Sun N., Panetta N.J., Gupta D.M., et al: Feeder-free derivation of induced pluripotent stem cells from adult human adipose stem cells. Proc Natl Acad Sci U S A 2009; 106: pp. 15720-15725
- 149. Staerk J., Dawlaty M.M., Gao Q., et al: Reprogramming of human peripheral blood cells to induced pluripotent stem cells. Cell Stem Cell 2010; 7: pp. 20-24
- 150. Loh Y.H., Hartung O., Li H., et al: Reprogramming of T cells from human peripheral blood. Cell Stem Cell 2010; 7: pp. 15-19
- 151. Zhao J., Jiang W.J., Sun C., et al: Induced pluripotent stem cells: origins, applications, and future perspectives. J Zhejiang Univ Sci B 2013; 14: pp. 1059-1069
- 152. Uto S., Nishizawa S., Takasawa Y., et al: Bone and cartilage repair by transplantation of induced pluripotent stem cells in murine joint defect model. Biomed Res 2013; 34: pp. 281-288
- 153. Diekman B.O., Christoforou N., Willard V.P., et al: Cartilage tissue engineering using differentiated and purified induced pluripotent stem cells. Proc Natl Acad Sci U S A 2012; 109: pp. 19172-19177
- 154. Ko J.Y., Kim K.I., Park S., et al: In vitro chondrogenesis and in vivo repair of osteochondral defect with human induced pluripotent stem cells. Biomaterials 2014; 35: pp. 3571-3581
- 155. Kim K., Doi A., Wen B., et al: Epigenetic memory in induced pluripotent stem cells. Nature 2010; 467: pp. 285-290
- 156. Feng R., Desbordes S.C., Xie H., et al: PU.1 and C/EBPalpha/beta convert fibroblasts into macrophage-like cells. Proc Natl Acad Sci U S A 2008; 105: pp. 6057-6062
- 157. Caiazzo M., Dell’Anno M.T., Dvoretskova E., et al: Direct generation of functional dopaminergic neurons from mouse and human fibroblasts. Nature 2011; 476: pp. 224-227
- 158. Ring K.L., Tong L.M., Balestra M.E., et al: Direct reprogramming of mouse and human fibroblasts into multipotent neural stem cells with a single factor. Cell Stem Cell 2012; 11: pp. 100-109
- 159. Vierbuchen T., Ostermeier A., Pang Z.P., et al: Direct conversion of fibroblasts to functional neurons by defined factors. Nature 2010; 463: pp. 1035-1041
- 160. Fishman V.S., Shnayder T.A., Orishchenko K.E., et al: Cell divisions are not essential for the direct conversion of fibroblasts into neuronal cells. Cell Cycle 2015; 14: pp. 1188-1196
- 161. Sekiya S., and Suzuki A.: Direct conversion of mouse fibroblasts to hepatocyte-like cells by defined factors. Nature 2011; 475: pp. 390-393
- 162. Szabo E., Rampalli S., Risueno R.M., et al: Direct conversion of human fibroblasts to multilineage blood progenitors. Nature 2010; 468: pp. 521-526
- 163. Efe J.A., Hilcove S., Kim J., et al: Conversion of mouse fibroblasts into cardiomyocytes using a direct reprogramming strategy. Nat Cell Biol 2011; 13: pp. 215-222
- 164. Ieda M., Fu J.D., Delgado-Olguin P., et al: Direct reprogramming of fibroblasts into functional cardiomyocytes by defined factors. Cell 2010; 142: pp. 375-386
- 165. Hiramatsu K., Sasagawa S., Outani H., et al: Generation of hyaline cartilaginous tissue from mouse adult dermal fibroblast culture by defined factors. J Clin Invest 2011; 121: pp. 640-657
- 166. Outani H., Okada M., Hiramatsu K., et al: Induction of chondrogenic cells from dermal fibroblast culture by defined factors does not involve a pluripotent state. Biochem Biophys Res Commun 2011; 411: pp. 607-612
- 167. Ishii R., Kami D., Toyoda M., et al: Placenta to cartilage: direct conversion of human placenta to chondrocytes with transformation by defined factors. Mol Biol Cell 2012; 23: pp. 3511-3521
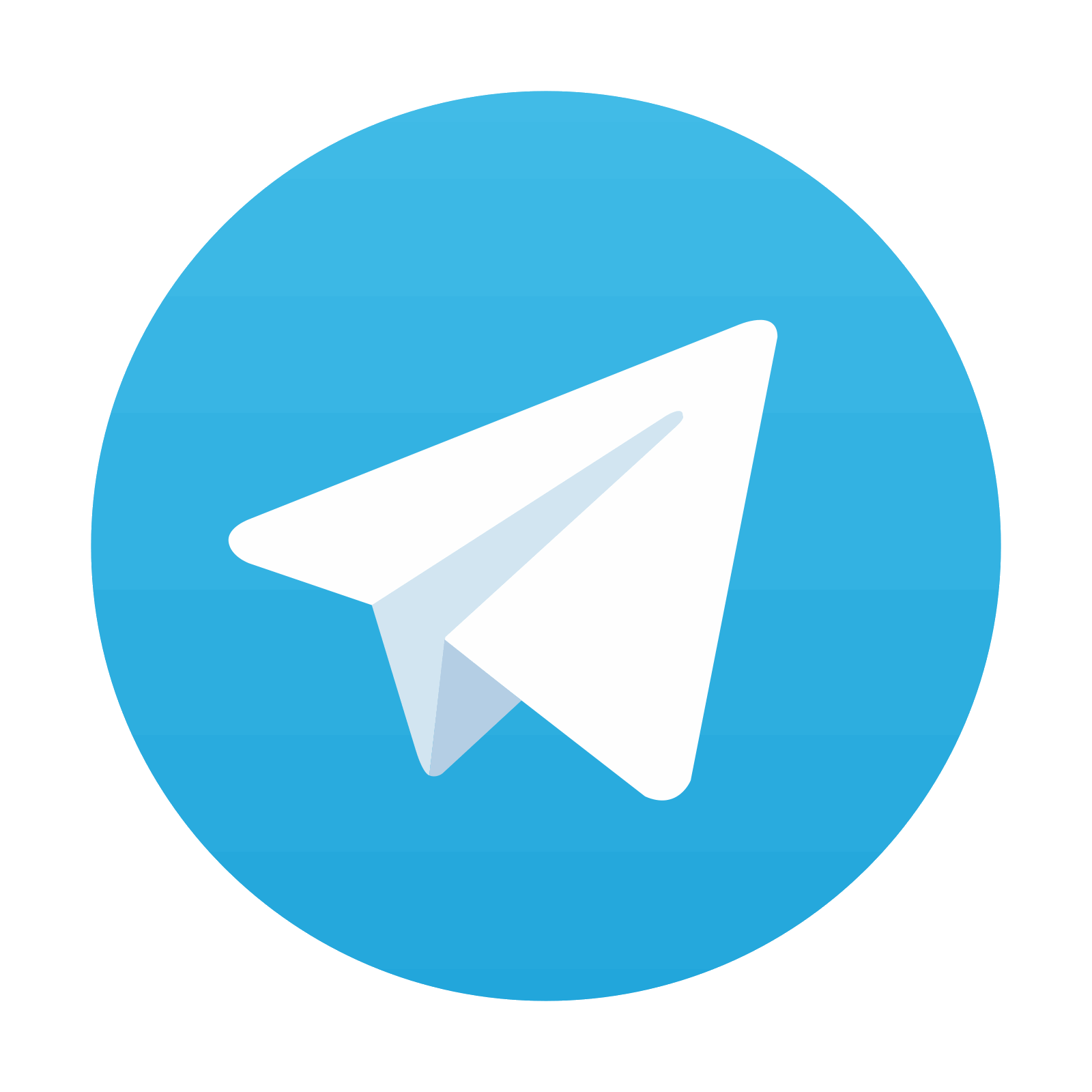
Stay updated, free articles. Join our Telegram channel
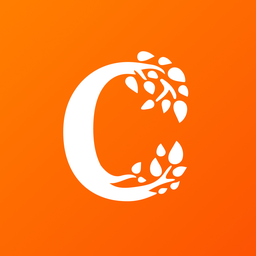
Full access? Get Clinical Tree
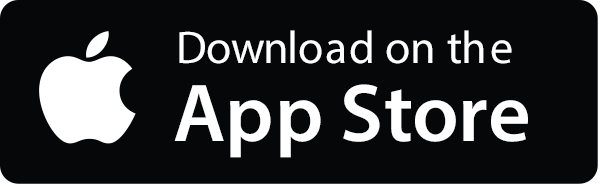
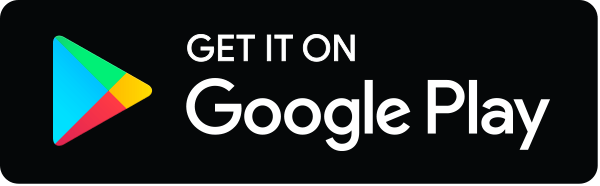