Pearls
- •
The anesthetic care of ICU patients is an extension of medical and anesthetic management principles employed in the operating room.
- •
Anesthesiologists caring for critically ill children must understand the desired therapeutic goals and each patient’s preexisting conditions.
- •
ICU physicians must be aware of the physiologic perturbations of anesthesia and surgery in pediatric patients to adapt management appropriately and understand the rationale for using particular agents.
- •
Understanding both long- and short-term anesthetic effects on various organ systems is still a growing field of research.
Anesthetic agents and regional anesthesia methods
Anesthetic agents alter normal homeostasis in patients with critical disease and/or trauma. These physiologic effects and consequences can be profound. Anesthetic agents have been shown to both be protective and detrimental to organ systems. An understanding of these effects is imperative to providing quality care for all patients, especially intensive care unit (ICU) patients. This chapter focuses on the most commonly used volatile and intravenous hypnotic and opioid medications. The use of local anesthetic agents and their clinical applications for pediatric ICU patients is also addressed.
Volatile or inhaled anesthetics are a key component of a balanced anesthetic regimen. The most frequently used inhaled agents are sevoflurane, isoflurane, and desflurane. While these all induce a state of unconsciousness and amnesia, each has distinct characteristics that may influence the selection of a particular agent for a specific clinical indication. Sevoflurane is the most commonly administered anesthetic for children. It can promote a rapid and smooth induction of anesthesia, as opposed to desflurane, which is a strong airway irritant that can cause coughing, laryngospasm, and hypoxemia. When comparing the anesthetic effects of inhalational agents, the concept of minimal alveolar concentration (MAC) is used. MAC is defined as the concentration that prevents movement in 50% of patients in response to a surgical incision. The use of MAC as a metric allows one to compare anesthetics of different potencies at a similar effect (MAC multiples) rather than comparing similar concentrations of drugs with differing potencies.
In addition to inhaled agents, balanced anesthesia includes the use of intravenous anesthetics such as propofol and dexmedetomidine. Propofol is a sedative hypnotic that is widely used as an induction agent in anesthesia as well as in continuous infusion for sedation in the ICU. It is rapidly distributed and cleared. Propofol is suspended in a lipid emulsion and a continuous infusion can result in a significant lipid load. Dexmedetomidine, a highly selective α 2 -adrenergic agonist, is a sedative analgesic used for both procedural and pediatric ICU sedation. It produces anxiolysis and analgesia via stimulation of α 2 -receptors in the brain and spinal cord and reduces the requirement for inhaled anesthetics and opioids. , Dexmedetomidine has a rapid distribution phase and an elimination half-life of 2 hours. , The pharmacokinetic profile in 2- to 12-year-old children is similar to that of adults, whereas clearance in 1- to 24-month-old infants is faster than in adults.
Because inhalational anesthetics can produce significant hemodynamic changes in compromised children, the use of high-dose opioids has been shown to confer hemodynamic stability and adequate anesthesia. , Frequently used opioids include fentanyl, remifentanil, and sufentanil. However, while high-dose opioid regimens promote hemodynamic stability, they delay neurologic recovery and cause respiratory depression. Opioids such as fentanyl and sufentanil, which undergo hepatic elimination, increase their terminal half-life with repeated doses. Remifentanil, a synthetic opioid, is metabolized by plasma and tissue esterases; thus, it has a more reliable pharmacokinetic profile, an extremely short half-life, and is not influenced by the duration of infusion. Fig. 128.1 depicts timing of decrease in site effect after termination of opioid infusion as a function of the duration of the infusion. Additionally, remifentanil clearance peaks in neonates and infants, and its terminal elimination half-life does not change with age. This is in direct contrast to other opioid agents—such as fentanyl, sufentanil, and morphine—which have the lowest clearance and longest elimination half-time during infancy. Thus, the pharmacokinetic and dynamic effects of remifentanil are more predictable than for the organ-eliminated opioids. Unfortunately, tolerance and hyperalgesia have been reported with remifentanil administration, limiting its potential use.

Local anesthetics and regional anesthesia are frequently employed components of anesthetic regimens. Local anesthetic use in regional anesthesia for children significantly improves perioperative pain and has the potential to decrease opioid consumption. Unlike in adults, most regional anesthetics in the pediatric population are performed under general anesthesia and with ultrasound guidance. This direct visualization of anatomy and local anesthetic infiltration has improved the quality of these nerve blocks. Ultrasound use has also decreased the incidence of nerve injury and decreased the amount of local anesthetic necessary to provide adequate pain control.
Local anesthetics are organized into two categories: amino esters and amino amides. Esters include cocaine, tetracaine, chloroprocaine, and prilocaine and are associated with allergic responses. These agents are metabolized by plasma and tissue esterases. Amide agents—which include bupivacaine, ropivacaine, levobupivacaine, and lidocaine—are more commonly employed for both central and peripheral nerve blockade in the pediatric population due to a more favorable toxicity profile. These agents are metabolized by the P-450 system within the liver. Duration of action is the shortest in neonates and infants.
Commonly performed blocks in the pediatric population include paravertebral, transversus abdominis plane (TAP), and the rectus sheath blocks. More recently, other types of nerve blocks, including blocks of the quadratus lumborum (QL) and erector spinae planes, are being performed. All of these regional anesthetics are considered peripheral nerve blocks and thus have fewer contraindications and complications than neuraxial blocks in critically ill patients.
The paravertebral nerve block can be performed in the setting of multiple rib fractures due to trauma or major thoracic or abdominal procedures. Local anesthetic is injected into the paravertebral space, resulting in blockade of the somatic and sympathetic nerves that exit at the level of injection ( Fig. 128.2 ). Recently, anesthetists have been performing erector spinae plane blocks instead of paravertebral blocks owing to their ease of placement. In this block, local anesthetic is injected under the erector spinae muscle, lifting it upward, resulting in a similar blockade ( Fig. 128.3 ).


Additional peripheral nerve blocks indicated for abdominal procedures include the TAP block, rectus sheath, and QL block. A TAP block involves local anesthetic injection between the internal oblique and transverse abdominis muscles where the thoracolumbar nerves lie, which is effective for laparoscopic procedures ( Fig. 128.4 ). More specifically, a rectus sheath block, where local anesthetic is injected between the posterior aspect of the rectus muscle and the posterior rectus sheath ( Fig. 128.5 ), can be used for procedures involving the umbilicus. Finally, a QL block is used for abdominal surgery below the umbilicus ( Fig. 128.6 ). At least three different needle approaches have been described, but no definitive evidence proves one more efficacious than another. Local anesthetic is injected in the plane surrounding the QL; the more specific location is determined by various needle approaches.




When employing regional anesthesia as either a primary anesthetic or adjunct to an anesthetic regimen, local anesthetic systemic toxicity (LAST) must be considered. LAST is a severe complication of local anesthetic use; children are at increased risk owing to lower concentrations of serum-binding proteins (α-1-acid glycoprotein and albumin). A slower rate of metabolism can also predispose children to LAST. This reaction is a combination of both neurologic and cardiovascular collapse, during which neurologic symptoms typically present first. , In the anesthetized patient, these symptoms can include muscle rigidity, increased blood pressure and heart rate, and seizure, followed by cardiovascular collapse. Prompt recognition and treatment with lipid emulsion is essential. An initial dose of 1.0 to 1.5 mL/kg (maximum, 10 mL/kg) 20% intralipid is followed by continuous infusion 0.25 to 0.5 mL/kg per minute in addition to any airway management or other supportive measures that are necessary.
Neurologic effects
The neurologic impact of anesthetic agents should be considered carefully in both healthy and critically ill patients throughout the perioperative setting. Traumatic brain injury is a leading form of pediatric trauma in the United States. , Measures to control intracranial hypertension, typically associated with poor outcomes, are employed throughout the perioperative period. Specifically, maintaining cerebral perfusion pressure is imperative to maintain neurologic function and is defined as the difference between mean arterial pressure (MAP) and intracranial pressure (ICP) or central venous pressure. Intracranial hypertension is defined as ICP greater than or equal to 15 to 20 mm Hg and increased systemic blood pressure is necessary to maintain cerebral perfusion. Signs of intracranial hypertension are listed in Table 128.1 . In patients with supratentorial lesions, 1 MAC of desflurane increased ICP, whereas an equal dose of isoflurane did not. This may be a result of increased cerebral spinal fluid production with the use of desflurane.
Infants | Children | Infants and Children |
---|---|---|
Irritability | Headache | Decreased consciousness |
Full fontanelle | Diplopia | Cranial nerve (III and VI) palsies |
Widely separated cranial sutures | Papilledema | Loss of upward gaze (setting sun sign) |
Cranial enlargement | Vomiting | Signs of herniation, Cushing triad, pupillary changes |
The most dynamic of the intracranial compartments (cerebral tissue, blood, cerebral spinal fluid) is blood volume, mediated primarily through cerebral vascular resistance. Factors that influence cerebral vascular resistance and cerebral blood flow (CBF) include arterial partial pressure of carbon dioxide (Pa co 2 ) and oxygen (Pa o 2 ), MAP, and various drugs, such as anesthetic agents ( Fig. 128.7 ). The brain maintains a constant CBF over a MAP ranging from 50 to 150 mm Hg in adults, known as cerebral autoregulation. This range likely shifts in concert with age-related changes in normal system blood pressures and cerebral perfusion pressures in infants and children. It may also shift in the setting of chronic hypertension, intracranial tumors, head trauma, or shock, thereby sensitizing the brain to ischemic effects. , CBF varies linearly by 2% to 4% for every 1 mm Hg of Pa co 2 ; anesthetized children exhibit greater vasoreactivity than adults. As Pa co 2 is lowered, cerebral vasoconstriction occurs, thus decreasing CBF. As it approaches 20 mm Hg, vasoconstriction can be significant enough to result in ischemia. This relationship is limited, and a diminished effect is seen over time. Pa o 2 less than 50 mm Hg results in cerebral vasodilation and increased CBF, whereas hyperoxemia exceeding 300 mm Hg may result in vasoconstriction.

Generally, inhaled anesthetics impair autoregulation and increase CBF. The effect of volatile anesthetics on autoregulation can be seen in Fig. 128.8 . Sevoflurane preserved autoregulation up to 1.5 MAC, whereas 1 MAC of desflurane impaired cerebral autoregulation, and at 1.5 MAC it was abolished. , Sevoflurane also better preserved autoregulation and had less of a vasodilatory effect than isoflurane. , In contrast, propofol at 200 μg/kg minute had no effect on autoregulation. Furthermore, the effect of Pa co 2 on CBF was more pronounced during isoflurane anesthesia than with sevoflurane. CBF values were measured via labeled magnetic resonance imaging (MRI) and found to be approximately 2.5 to 3 times lower in most regions during a fentanyl anesthetic versus isoflurane. However, opioid anesthetics in conjunction with propofol have been shown to maintain cerebral autoregulation.

Cerebral metabolism—specifically, the cerebral metabolic rate of oxygen (CMRO 2 ), is also affected by anesthetic agents. Volatile agents “uncouple” the normal relationship between CBF and CMRO 2 , resulting in increased vasodilation. However, isoflurane appears to decrease CBF with associated hyperventilation to a Pa co 2 to 20 to 25 mm Hg. In contrast, nitrous oxide is known to cause cerebral vasodilation and increase the CMRO 2 , effects that may be countered by the addition of intravenous agents. Sevoflurane has been shown to increase CBF and ICP while decreasing the CMRO 2 . Desflurane and isoflurane have similar effects on CBF and exhibit burst suppression at 1.24 MAC. Propofol produces global metabolic depression and decreased CMRO 2 , CBF, and ICP while preserving the response to arterial CO 2 . ,
Intravenous agents are also able to modify CBF and cerebral metabolism. When measuring cerebral tissue oxygenation, MAP, heart rate, Pa o 2 , Pa co 2 , hemoglobin, and middle cerebral artery (MCA) mean flow velocity at various time points following a bolus administration of 3 mg/kg of propofol, cerebral tissue oxygenation and MCA flow velocity were shown to decrease without significant decrease in the other end points. In a study comparing the effects of dexmedetomidine, sevoflurane, and propofol on cerebral metabolism in 150 healthy male subjects, dexmedetomidine and propofol decreased cerebral metabolism most significantly. This is an important finding when considering possible dexmedetomidine-induced cerebral vasoconstriction or cerebral hypoperfusion related to anesthesia or surgical procedure. Further study comparing dexmedetomidine and propofol revealed that dexmedetomidine provided deeper sedation during deep brain stimulation while maintaining cerebral perfusion pressure, CBF velocity, and regional brain oxygenation. In contrast to the uncoupling of CBF and metabolic rate demonstrated by volatile agents, dexmedetomidine was found to preserve CBF-CMR coupling due to a more significant decrease in cerebral metabolism compared with blood flow velocity. The effects of multiple anesthetic agents on CBF and CMRO 2 are depicted in Fig. 128.9 .


Anesthetic agents have variable effects on other neurologic effects, such as emergence delirium. Sevoflurane is associated with a high incidence of emergence delirium. Significant differences were found in frontal lobe functional connectivity between children with and without emergence delirium after discontinuation of sevoflurane. Furthermore, electroencephalographic (EEG) patterns in children without emergence delirium transitioned from an indeterminate state to classifiable sleep before peaceful awakening, whereas patients with emergence delirium demonstrated EEG changes during that indeterminate state. Sevoflurane has also been associated with epileptiform activity in pediatric patients with or without preexisting seizure disorders. ,
Concerns have also been raised regarding anesthetic agents and their toxic neurocognitive effects on the developing brain. Animal studies have demonstrated objective findings with respect to an increase in apoptosis in cortical and subcortical tissue, decreases in dendritic spine formation, decreases in synapses, and alterations in neurogenesis with respect to proliferation of neuronal precursor cells, their differentiation into neuron glia, and their migration and functional integration into the neuronal circuitry. Various anesthetic agents have been demonstrated to produce these findings when used either as single agents or in combination. Animal studies have shown an age window of vulnerability that appears to be species specific. Dose and duration of exposure have also been associated with these neurocognitive impairments. Of note, in some animal studies, the neurotoxic effect can be reversed when the animals are placed in an enriched environment, suggesting that this neurocognitive impairment can be attenuated. Recent animal studies have alluded to the possible neuroprotective effects of dexmedetomidine. , In a study examining the brains of sheep that underwent surgery at 118 to 120 days’ gestational age with 1.5% to 2.0% isoflurane for 2 to 3 hours and a subsequent 6-hour procedure 2 weeks later, concurrent dexmedetomidine administration revealed decreased hippocampal neuroapoptosis compared with the isoflurane-only group. Furthermore, dexmedetomidine mitigated sevoflurane-induced cell cycle arrest and inhibitory factors in neonatal rat hippocampal cells. A systematic review of 20 animal studies evaluating neuroprotective and neurobehavioral effects of dexmedetomidine confirmed histologic injury in 3 of 11 studies but revealed decreased injury after a previous anesthetic in 13 of 16 studies. Additionally, neurobehavioral tests were performed in 7 of the 20 studies, 3 of which tested the effects of dexmedetomidine alone and 6 following a prior anesthetic. Dexmedetomidine had no negative effect on neurobehavioral testing when used alone and was found to lessen the negative effects induced by a previous anesthetic. Though animal studies have been quite suggestive of anesthetic neurotoxicity, human data are less definitive. Wilder et al. at the Mayo Clinic in Rochester, Minnesota, compared the incidence of learning disabilities in children anesthetized before the age of 4 years to children who had not undergone anesthesia. In this article, the incidence of learning disabilities in children with a single exposure was the same as those who did not have an anesthetic exposure. However, in patients with greater than one anesthetic exposure, the incidence of learning disabilities was almost two times greater. In more recent studies of children with a single anesthetic exposure, Sun et al. conducted a bidirectional study of children undergoing inguinal hernia repair before age 3 years and reported no difference in intelligence quotient (IQ) when these patients were compared with their siblings who had not been exposed to anesthesia. In a multi-international center study (GAS) of children undergoing hernia repair in infancy anesthetized with either general anesthesia or administered an awake-regional anesthetic, McCann noted no difference between the groups with respect to a full-scale IQ on the Wechsler Preschool and Primary Scale of Intelligence, third edition (WPPSI-III), at 5 years of age. In the Mayo Anesthesia Safety in Kids (MASK) study, Warner used propensity matching from a population-based cohort of children undergoing a variety of surgical procedures and reported no effect on full-scale IQ.
A few studies have reported on the effects of multiple anesthetic exposures before 5 years of age as well as age of exposure with regard to neurotoxicity vulnerability. The end points used in these studies were different; consequently, comparison of results is difficult. Glatz, in a large-scale study of children in Sweden, noted a small, insignificant difference in school grades and IQ test scores. The magnitude of the difference was the same after multiple exposures, and age of exposure did not appear to be a factor. However, differences in outcome were more affected by sex, maternal educational level, or month of birth during the same year.
O’Leary conducted a large-scale study of Canadian schoolchildren and noted that children 2 years old or older at the time of their first surgery had increased odds of an early developmental vulnerability compared with unexposed children (odds ratio, 1.05; 95% confidence interval, 1.01–1.10). There was no increase in odds of early developmental vulnerability with increasing frequency of exposure.
Currently, much remains to be determined with respect to specific anesthetic agents, threshold values for toxicity with regard to dose, number of exposures, patient age vulnerability, and patient risk factors.
Cardiovascular effects
Historically, inhalational anesthesia was associated with a higher rate of cardiac arrest, bradycardia, and hypotension in infants and children than in adults; these effects were noted to be dose dependent. Many of these effects can be attributed to the use of halothane, which is no longer commonly used. While isoflurane was associated with reduction in systemic vascular resistance, contractility, and blood pressure, cardiac index was better preserved in comparison with halothane. At equipotent concentrations (1 MAC), desflurane and isoflurane attenuate the baroresponse in children. The hemodynamic profile of sevoflurane in children is similar to isoflurane.
In a retrospective study of 3548 congenital heart disease (CHD) patients undergoing cardiac MRI under general anesthesia (GA), cardiac index (CI) and left ventricular ejection fraction (LVEF) were measured and compared with a subset of patients within the CHD group who were sedated as well as healthy controls. Mean CI was significantly lower in the GA group without significant change in LVEF. Compared with awake values, anesthetized children had a 30% decrease in arterial blood pressure at 1 MAC of desflurane with a minimal change in heart rate. Rapid increases in desflurane resulted in transient increases in arterial blood pressure and heart rate attributed to both increased sympathetic and renin angiotensin system activity.
Cardiovascular adverse effects related to mechanical ventilation strategies were demonstrated in rats using biomarkers such as B-type natriuretic peptide (BNP), vascular endothelial growth factor (VEGF), and endothelin-1 (ET-1). BNP increased with both high and low positive end-expiratory pressure (PEEP) compared with ventilated controls. VEGF was influenced by high PEEP, hyperoxemia, hypoxemia, and hypocapnia, whereas a change in ET-1 was found only in the setting of hypoxemia. This indicates that various mechanical ventilation strategies may adversely influence both the cardiovascular and respiratory systems.
Intravenous anesthetic agents have frequently been employed for maintenance of hemodynamic stability, specifically opioids. A more variable response is noted with dexmedetomidine and a typically negative effect with propofol administration. A study of premature infants undergoing patent ductus arteriosus ligation reported the safety and efficacy of fentanyl anesthesia. Similarly, high-dose fentanyl (50–75 μg/kg) and sufentanil (5–10 μg/kg) in children with CHD decreased heart rate by only 7% and MAP by 9%. Furthermore, pulmonary vascular resistance decreased as well.
Dexmedetomidine produces a biphasic blood pressure response, an initial increase in blood pressure followed by a decrease, which returns to baseline within minutes. Heart rate, however, remains low with bolus dexmedetomidine. This response is attributed to the initial stimulation of α 2b -receptors, resulting in vasoconstriction and, subsequently, a more powerful response at the α 2a -receptors, which causes sympatholysis. A bolus dose of 0.5 μg/kg dexmedetomidine administered to patients undergoing endomyocardial biopsy caused an increase within 1 minute in systolic and diastolic blood pressure, systolic and diastolic pulmonary artery pressure, pulmonary artery wedge pressure, and systemic vascular resistance but returned to baseline by 5 minutes after injection. Only heart rate decreased following injection and remained lower after 5 minutes. No significant changes were noted in central venous pressure or pulmonary vascular resistance. The heart rate and blood pressure of 17 pediatric patients on a continuous infusion of dexmedetomidine were analyzed at various points. No cardiac conduction abnormalities were found. However, a 20% decrease in heart rate from baseline occurred in 35% of patients, but this was not statistically significant over time. Only a single patient required discontinuation of infusion due to low heart rate. Blood pressure changes were variable, with a statistically significant increase in systolic blood pressure by 0.4 mm Hg/h of infusion. The most common adverse effects of dexmedetomidine are hypotension and bradycardia, which are more significant in the setting of underlying cardiac disease or use in conjunction with other negative chronotropic agents (propofol, succinylcholine, digoxin). Bloor et al. noted no hemodynamic response to a 0.7 μg/kg per hour infusion of dexmedetomidine, but a 1 μg/kg bolus dosing resulted in an increase in MAP and a decrease in heart rate. Bolus doses of 0.25, 0.5, 1.0, and 2.0 μg/kg were administered to healthy males; MAP decreased 14%, 16%, 23%, and 27%, respectively. Following a 1 μg/kg bolus dose, cardiac output decreased by 20% within the first minute but returned to 90% of baseline by 60 minutes. A 2 μg/kg bolus dose resulted in a 60% decrease in cardiac output that returned to 85% of baseline within 60 minutes.
In 30 infants and children undergoing cardiopulmonary bypass randomized to receive dexmedetomidine (1 μg/kg load, 0.5 μg/kg per hour infusion) or placebo, plasma cortisol, norepinephrine, epinephrine, and glucose levels were significantly lower in the dexmedetomidine group. Additionally, dexmedetomidine has been reported to have antiarrhythmic effects in the perioperative period and may be useful for terminating reentrant supraventricular tachycardia. ,
In contrast, propofol has a well-described cardiac depressant effect. Induction doses of propofol (2–3 mg/kg) can result in a 10% to 15% decrease in MAP as well as bradycardia. There is a modest negative inotropic effect due to antagonism of β-adrenergic receptors and calcium channels. Prolonged infusions of greater than 48 hours and rates of 4 mg/kg per hour have been associated with propofol-related infusion syndrome (PRIS). The syndrome is characterized by severe bradycardia resulting in heart failure, metabolic acidosis, hyperlipidemia, rhabdomyolysis, and subsequent hyperkalemia and renal failure. Pediatric patients appear to be more susceptible than adults to PRIS due to low glycogen stores and need for fat metabolism. Risk factors for PRIS include respiratory failure, traumatic brain injury, or other critical illness. Triggering agents such as catecholamine and steroid infusion have also been associated with the syndrome. This reaction has been attributed to mitochondrial electron transport inhibition and impaired oxygen utilization with subsequently decreased ATP production, mitochondrial lipid metabolism, and accumulation of arrhythmogenic and toxic long fatty acid chains. , PRIS management requires prompt recognition and discontinuation of the infusion with aggressive supportive care, including inotropes, fluids, pacing, hemodialysis, and even extracorporeal membrane oxygenation. , ,
Respiratory, gastrointestinal, and renal effects
While evidence of neurologic and cardiovascular effects is certainly more vast, anesthetic agents are able to modify other organ systems as well. As previously mentioned, potential adverse respiratory effects related to mechanical ventilation technique were demonstrated by altered levels of BNP, VEGF, and ET-1. Furthermore, significant increases in airway resistance have been reported in children with known reactive airway disease when anesthetized with desflurane. In a study comparing desflurane and sevoflurane with laryngeal mask airway, respiratory events were similar. However, more children experienced laryngospasm and mild desaturation in the desflurane group. Children undergoing MRI experienced significantly less frequent adverse airway events with a propofol anesthetic versus isoflurane. Dexmedetomidine appears to have minimal effect on respiration and may be useful in the setting of upper airway obstruction.
In a systematic review of randomized controlled trials comparing general anesthesia with volatile anesthetic agents or intravenous agents, the occurrence of postoperative nausea and vomiting was significantly less with intravenous agents. A study of 150 children demonstrated that pH parameters (number of reflux episodes >5 minutes, duration of longest reflux episode, time pH <4 and fraction of time pH <4) significantly increased in the first hour after anesthesia. Furthermore, epidural lidocaine was compared with epidural saline in rats in the setting of intestinal ischemia and subsequent ileus. This study found that rats who received epidural lidocaine resolved their ileus much more rapidly than those that did not, indicating that epidural lidocaine not only aids in the treatment of ischemic pain but also in recovery of function.
In a review of 32 published reports of surgical patients, the evidence based on basic measures of renal function, BUN, and creatinine indicated an absence of renal toxicity during sevoflurane anesthesia. Sevoflurane-induced renal toxicity due to its fluoride metabolite was unlikely in a study in which fluoride levels were measured in children after sevoflurane anesthesia. Sevoflurane anesthesia over a period of 4 hours in pediatric patients with normal renal function produced compound A, a known renal toxin, in concentrations of 15 ppm or less. These patients showed no evidence of abnormal renal function up to 24 hours.
In a meta-analysis of postoperative pediatric cardiac patients, dexmedetomidine was associated with a significantly lower incidence of acute kidney injury (AKI). Intraoperative infusion of dexmedetomidine was also associated with less AKI, measured by estimated glomerular filtration rate and serum creatinine, than in control groups who did not receive dexmedetomidine during pediatric cardiac surgery. Finally, dexmedetomidine decreases renin and vasopressin levels, promotes diuresis, and reduces sympathetic tone.
In summary, the anesthetic care of ICU patients requires that both intensivists and anesthesiologists have an intimate knowledge of intensive care and anesthetic principles and methods employed throughout the perioperative setting. Specific therapeutic goals can only be achieved with these principles in mind and an understanding of a critically ill patient’s ongoing pathology and preexisting conditions. Anesthetic agents have a multitude of effects on several organ systems ranging from benign or fatal cardiovascular changes to potential neurotoxic or neuroprotective impacts. This chapter reviewed several of the most commonly used anesthetic agents throughout the perioperative period and the expansive impact that they may have on the critically ill patient.
Key references
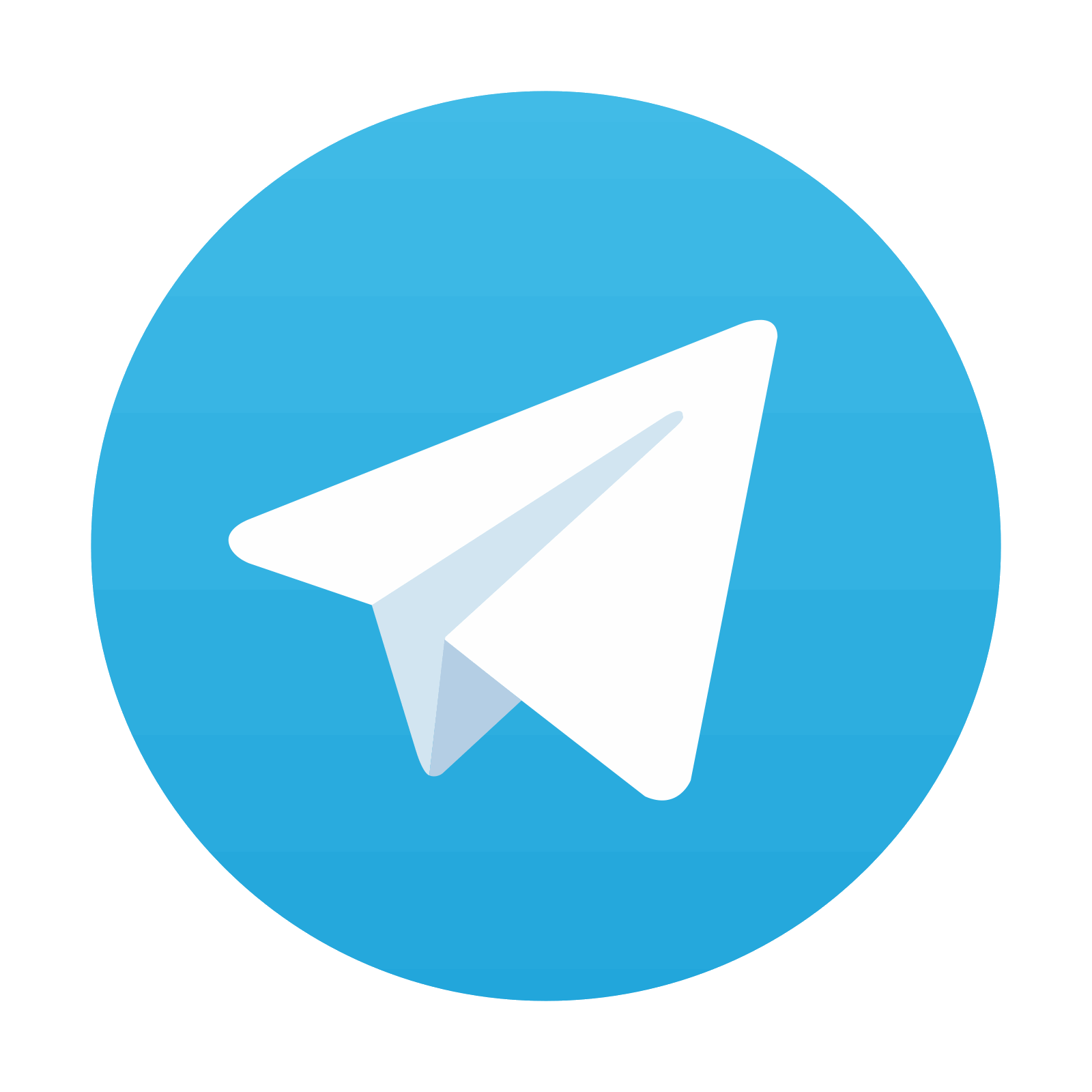
Stay updated, free articles. Join our Telegram channel
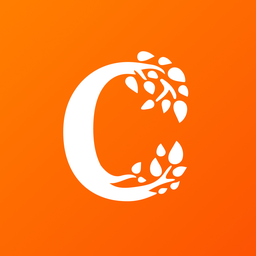
Full access? Get Clinical Tree
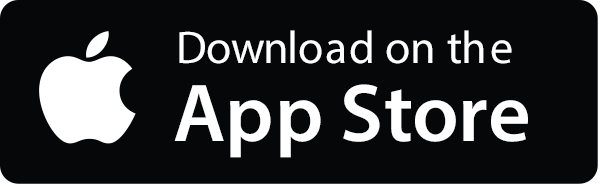
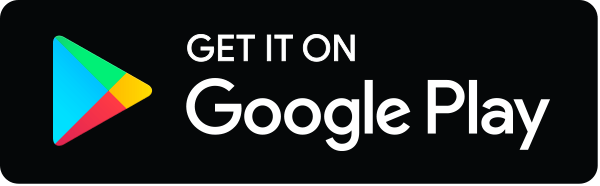
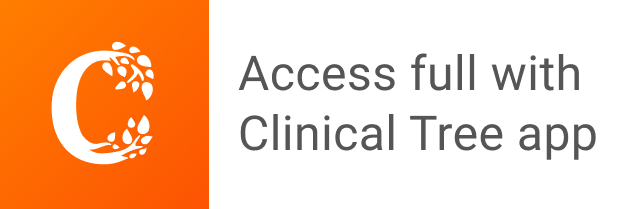