Introduction
Allograft tissues are obtained from a donor source and transplanted into a different recipient or host, while autologous tissues are obtained and redirected into the same individual. Allograft tissue has been used in interventional orthopedics to provide structural support, act as a scaffold for the growth of new tissue, or initiate and regulate the body’s innate healing response. Allografts offer several advantages over autografts. Use of an autologous tissue requires harvesting of the blood, bone, bone marrow, or adipose tissue, which can be painful, require additional time to process the tissue, and carry procedural risks not associated with allografts. Moreover, allograft tissues can provide patients with access to orthobiologic treatments when autologous are not feasible, such as in the setting of systemic comorbidities or when pharmacologic interactions may not allow for autologous harvesting.
The potential therapeutic benefits of allogenic tissues are apparent; however, their true cellular composition and role in clinical practice is still debated. Furthermore, potential risks of cellular allografts include contamination with bacteria during tissue processing or graft rejection as a result of the allograft’s donor cell population persisting. The greatest risk of cell-based treatments is graft versus host disease. In large-scale allograft transplantation, a chimeric cell population has been noted in soft tissue transplants and osteochondral allograft transplantation. Fortunately, in some applications, the cellular allograft elements may exert their beneficial effects and subsequently be cleared from the recipient. The risk of disease is always possible when transplanting tissue from one source to another, and although rare, this possibility must always be considered when evaluating potential allograft products for clinical use.
The goal of this chapter is to review the basic science, clinical applications, and logistic considerations of several allograft tissues within the spectrum of interventional orthopedic procedures.
Cellular Versus Acellular Allografts
Allografts in interventional orthopedics should be categorized as either cellular or acellular. For musculoskeletal purposes, cellular allografts theoretically contain living nucleated cells, including leukocytes, hematopoietic stem cells, or mesenchymal stem cells (MSCs), in addition to platelets, growth factors, and other cytokines. These tissues are typically derived from blood, bone marrow, adipose, or placental tissues. The MSC has traditionally been the focus of cellular allografts for interventional orthopedics. Outside of the United States, clinicians are allowed to isolate and expand cells like MSCs in culture prior to transplantation into a patient. Culture-expanded products contain a greater concentration of MSCs than their nonexpanded counterparts; however, this practice is not currently allowed within the United States per Food and Drug Administration (FDA) regulations and is considered more than “minimal manipulation.” Per current FDA regulations, use of biologic tissues must fall under the Human Cells, Tissues, and Tissue Based-Products (HCT/Ps) guidance reports :
|
|
|
|
|
|
|
|
|
Acellular allografts are those that have been irradiated or processed in such a manner where the product contains no nucleated cells or the cells that are present are no longer viable. These products rely on growth factor and cytokine content to mediate the healing processes. The presence of various growth factors and cytokines can be tested by performing enzyme-linked immunosorbent assay (ELISA) and further confirmed with tests such as a Western blot analysis. Acellular allografts, like demineralized bone matrix (DBM), also have the capability to provide structural support or act as a scaffold for the proliferation of cellular components.
Placental-derived products (amnion, cord blood, Wharton jelly) and exosome preparations characterize this subset of acellular allogenic products. While these tissues are known to have or be associated with MSCs in vivo, commercial processing techniques have been shown to negatively affect the viability of these cells. Therefore, even though these products possess the potential to stimulate healing, they should not be considered “stem cell therapies.”
Despite being acellular, there are many growth factors and cytokines that are relevant to interventional orthopedics. Growth factor and cytokine concentrations present in the allograft tissue may vary by the host donor tissues utilized. Additionally, the concentrations of growth factors and cytokines may also be altered by the time between tissue acquisition and processing, and administration by the clinician. Highlighted below are just some examples of these biomolecules along with their respective roles and functions.
Bone morphogenetic protein-2 (BMP-2)
- •
Induces chondrogenic and osteogenic differentiation
- •
Vital in regulating cell interactions
- •
Role in cartilage restoration has been theorized, but not thoroughly proven (fully elucidated
Platelet derived growth factor-β (PDGF-β)
- •
Accelerates extracellular matrix deposition and collagen formation
- •
Upon injury, stimulates influx of inflammatory factors and fibroblasts
Transforming growth factor-β1 (TGF-β1)
- •
Important mediator of tissue repair
- •
Released by platelets during acute injury response
- •
Influx to a wound is critical for macrophage and fibroblast chemotaxis
Interleukin-8 (IL-8)
- •
Induces neutrophil chemotaxis
- •
Increases vascular endothelial growth factor expression
- •
Secreted by monocytes in response to inflammatory stimuli
Vascular endothelial growth factor
- •
Potent stimulator of angiogenic cascade
- •
Regulates endothelial migration and proliferation
- •
Induces vascular permeability for increased nutrient delivery
- •
Promotes epithelization
The Mesenchymal Stem Cell
The name “mesenchymal stem cell,” first coined in 1991, has undergone multiple iterations. This is due, in part, to the fact that upon transplantation in clinical practice the cell may differentiate into the target tissue, but it is unclear whether the cell retains its stem capabilities. Recently, terms like “connective tissue progenitor cell,” “mesenchymal stromal cell,” and “medicinal signaling cell” have been offered as successors to the term “mesenchymal stem cell” in hopes of more accurately representing the true capabilities and biology of this type of cell. As the terminology and precise role of these cells in clinical practice remains a topic for debate, we will utilize the traditional name “mesenchymal stem cell” (MSC) for the purposes of this chapter. The specific name notwithstanding, the International Society of Cellular Therapy (ISCT) has offered explicit criteria that must be met in order to be considered an MSC ( Table 8.1 ).
|
|
|
A tissue sample can be evaluated for MSCs by identifying nucleated cells using a hemocytometer or cell counter. The mere presence of cells in a sample does not indicate viability; fluorescent dyes and various assays need to be used to properly determine a cell’s viability. Even so, clinicians should pay specific attention to the type of test and fluorescent marker used, as some dyes only indicate a cell’s presence without regard to whether the cell is alive or dead. If viable cells have been identified, additional testing must be performed to confirm the presence of MSCs based on the definition put forth by the ISCT (see Table 8.1 ). Simply assuming MSCs are present based on the expression or lack of expression of a single or few of the cluster of differentiation (CD) surface molecules can lead to an improper determination of MSC content in a given product.
MSCs are derived from various sources, either autologously or allogenically, and have been employed in animal and human studies for a variety of conditions in the nonoperative setting and as a surgical adjunct. MSCs are perivascular cells that, when activated in response to injury, migrate to the damaged site and serve as the main repair modulator of the musculoskeletal system. Upon arrival, they secrete various trophic and immunomodulatory factors that direct the body’s healing response. Clinically, isolating and applying multipotent MSCs can assist, or even generate, a healing cascade.
Autologous MSCs derived from bone marrow and adipose have been used to treat osteoarthritic conditions, particularly knee osteoarthritis ; however, autologous MSCs used in the setting of tendinopathies, bone pathologies, and other soft tissue injuries have also garnered attention. Application of allogenic MSCs and other biologics mimic those described in autologous studies, but a paucity of human studies using these products are present in the literature. There are numerous studies using animal models for the treatment of various muscular and tendon pathologies ; however, degenerative joint disease is the most thoroughly studied.
In the few human trials available, the focus has been on osteoarthritis and these studies have demonstrated good results. Allogenic tissues have also been used as surgical adjuncts in spinal fusions and osteochondritis dissecans (OCD). The current literature for other soft tissue pathologies, including lateral epicondylitis, plantar fasciitis, Achilles tendinopathy, and meniscal injury is limited, but the initial studies are promising. There is still a great degree of research to be done to properly evaluate the true potential of allogenic biologic therapies and to identify whether each donor source carries the same therapeutic potential.
Culture-Expanded Allografts
Culture-expanded allografts are an attractive source of MSC, due to the ability to deliver a homogenized product with a high yield of MSCs. MSCs have classically been derived from autologous bone marrow or adipose tissue; however, patients are not always amenable to these types of harvests. Autologous aspirations may not be possible for patients with significant comorbidities and may yield a collection with a heterogeneous cell population with varying volumes of MSCs. Allogeneic sources offer numerous advantages. Cells can be derived from bone marrow and adipose, as well as placental tissues like the amnion, chorion, or umbilical cord. The opportunity to expand cells prior to administration and deliver a standardized, homogeneous product with low immunogenic properties makes MSCs isolated from allogeneic sources an attractive therapeutic option.
MSCs can be isolated from various portions of the umbilical cord: Wharton jelly, umbilical cord blood, or the umbilical cord as a whole. Umbilical cord blood has been shown to yield fewer total MSCs than those isolated from bone marrow, adipose tissue, Wharton jelly, or umbilical cord matrix. Furthermore, isolation efficiency is significantly lower when attempting to expand MSCs from umbilical cord blood compared to the other sources. , , Umbilical cord matrix MSCs have demonstrated faster doubling times than bone marrow isolates, as well as displaying minimal to no deterioration in division and growth power, or senescence, after multiple cell expansion passages. , Reports on the proliferative capacities of various tissue sources are conflicting and continue to be debated in the literature. , , , ,
As MSCs can be derived from different sources, it must be considered whether these various isolates carry the same biologic potential. It has been well established that MSCs expanded from adult and neonatal tissues have the capacity for chondrogenic differentiation; however, the capability for osteogenic and adipogenic differentiation is less consistent across cell sources. MSCs derived from adult bone marrow or adipose tissue have been thoroughly established to have tri-lineage differentiation, , , and cells isolated from Wharton jelly have been shown to exhibit similar differentiation capacities to adult bone marrow. , Umbilical cord matrix MSCs have also proven capable of tri-lineage differentiation. MSCs expanded from the amniotic membrane have displayed poor adipogenic and superior osteogenic differentiation capabilities compared to those derived from the chorionic plate, which have exhibited superior adipogenicity and poor osteogenicity. , Umbilical cord blood-derived MSCs represent the most conflicting source of cells. It has been confirmed these MSCs have the capability of adequate osteogenic and chondrogenic differentiation, with reports of reduced matrix formation, but their capacity for adipogenicity is debated in the literature ( Table 8.2 ). , , ,
Tissue Source | Chondrogenic | Adipogenic | Osteogenic |
---|---|---|---|
BM | + | + | + |
AT | + | + | + |
WJ | + | + | + |
AM | + | − | + |
CP | + | + | − |
UCB | + | ± | + |
Neonatal tissues have been suggested to be the optimal sources of MSCs compared to adult tissues due to a theoretically greater capacity for expansion, differentiation, and engraftment. , Ultimately, Wharton jelly may be the most promising source of MSCs for musculoskeletal tissue engineering due to its cellular yield, immunocompatability, proliferative capacity, and noninvasive collection procedure. ,
Cellular expansion has classically been completed in a two-dimensional (2D) monolayer characterized by repeated culturing of stem cells in plates or flasks. These methods ultimately lead to cell senescence and diminished multipotent capabilities. As such, three-dimensional (3D) culture systems have been developed, including perfusion cell systems, rotatory culture systems, stirred suspension systems, and microcarrier systems. These 3D expansion techniques are simple, reproducible, efficient, and offer advantages not observed in 2D systems. 3D cultures more favorably mimic the in vivo microenvironment of stem cells and ultimately increase their therapeutic potential. These systems are not influenced by substrate attachments as heavily as 2D systems, which improves cellular fortitude and differentiation capacity. , Additionally, they exhibit numerous advantages at the genomic level. , Expression of OCT4, SOX2, NANOG, and C-MYC is better maintained in 3D culturing systems than 2D. , These core transcription factors develop networks that preserve stem cells’ pluripotent state and capacity for self-renewal, are found in various sources of MSCs, and gradually disappear as cells are continuously cultured. , MSCs isolated from bone marrow have been shown to demonstrate a greater expression of these transcription factors than Wharton jelly or umbilical cord blood.
While there are studies that show that higher quantities of autologous MSCs yield better clinical results, this premise has not yet been substantiated. It is theorized that allogenic cells may be immune evasive, but not immune privileged and after transplantation eventually get removed by the host immune system. The closer a donor is to a human leukocyte antigen (HLA) match the more likely cells are to survive. Their potential therapeutic effects may be reduced as they have less time to secrete their paracrine factors. It would be unlikely that these allogenic cells could differentiate successfully to target tissue, as they may be removed by the host prior to differentiation. , Furthermore, clinical evidence supporting the use of culture-expanded autologous or allogenic MSC grafts over nonculture-expanded MSC grafts for musculoskeletal disease is not currently available. It is worth again emphasizing that culture-expansion of MSCs is not currently allowed in clinical practice in the United States.
Placental-Derived Allografts
Amniotic Tissue
The placental membrane is composed of the outer chorion and the thin, innermost amniotic membrane ( Fig. 8.1 ). The amniotic membrane, in conjunction with the amniotic fluid, envelop the developing fetus and provide nutritional, immunomodulatory, and structural benefits. Stem cells present in the amniotic membrane contribute and interact with the developing innate and adaptive immune systems in part by hindering leukocyte differentiation into dendritic cells and diminishing T-cell proliferation. The amniotic membrane and fluid also have numerous antimicrobial and antiviral effects. , The amniotic membrane and amniotic fluid have been identified in vivo as substantial sources of pluripotent and MSCs, and are considered advantageous due to minimal ethical concerns regarding the harvest of these tissues. , Cells derived from amniotic tissues have been used to treat human skin wounds, induce corneal reepithelization, and promote neochondrogenesis and peripheral nerve regeneration. , The pluri- and multipotent potential of these cells have made amniotic tissues attractive sources of MSCs in orthopedic tissue regeneration. ,

While the amniotic membrane can be used intraoperatively in the form of tissue sheets, the membrane can also be lyophilized and pulverized to particulate matter and used as an injectable. These products are often a combination of amniotic membrane and umbilical cord tissue and have functioned as an anti-inflammatory, anti-scarring, and regenerative agent. A primary therapeutic efficacy of amniotic membrane-umbilical cord tissue is due to its unique biochemical complex: heavy chain-hyaluronan-PTX3 (HC-HA/PTX3). This complex assists in the promotion of macrophage phagocytic activity, neutrophil apoptosis, , and suppression of TGF-β activity. , The amniotic membrane-umbilical cord products are received in a solid state. These can be stored at room temperature in the office, have a longer shelf life, and are reconstituted with saline prior to transplantation.
Amniotic fluid is typically harvested at the time of birth or in the second trimester, and is known to contain a heterogeneous population of cells in vivo, including MSCs. , , , The composition of the amniotic fluid varies dependent on the gestational age at the time of harvest, which likely affects the therapeutic potential of the tissue. Typically, the commercial fluid preparations are cryopreserved at −80°C and are shipped to the provider overnight in dry ice. Upon receipt, the product must be thawed out and transplanted into patient. Alternatively, the clinic may store the product in a freezer at −80°C until ready for use.
Umbilical Cord Blood
Umbilical cord blood is a rich source of progenitor cells that has the potential to be used as a therapeutic. Hematopoietic progenitor and stem cells from cord blood have a high recovery efficiency even after years of cryopreservation. First used to treat hematologic malignancies, umbilical cord blood is currently being explored for orthopedic applications. Cord blood has been employed in surgical cartilage repair with promising results when used in conjunction with a hyaluronate hydrogel, allowing clinicians to theorize about its use in nonsurgical interventions. The main limitations of the use of cord blood are the current need for HLA matching as well as the risk of graft versus host disease.
Wharton Jelly
Wharton jelly, the connective tissue of the umbilical cord, has also gained increasing interest as a potential source of stem and progenitor cells. Compositionally, Wharton jelly is agreed to have progenitor populations in the various tissue layers; however, there is no consensus on whether the cells in the regions are phenotypically identical. , It has been postulated that the MSCs derived from Wharton jelly originate as perivascular cells, but recent literature has brought this assertion into question. Similar to umbilical cord blood, Wharton jelly as a source of MSCs is currently being examined in interventional orthopedics. There are currently registered clinical trials underway for its use for a variety of orthopedic conditions.
The true capacity for these tissues to deliver MSCs as commercial products have come into question as it is unclear whether commercial processing, including tissue preservation and storage, affects the viability of cells in the products. , In an analysis of commercial amniotic fluid products, it was found that those advertised as “stem cell” and cellular products either did not contain any cells, or if cells were present, were unable to be cultured to an appreciable level. Known to be a potent source of growth factors and cytokines in vivo, the products were found to retain a number of these biomolecules—although the specific proteins present and volume of each varied across products. Such studies indicate that the therapeutic potential of allogenic amnion-derived products likely does not depend solely on cellular viability. , While the content of MSCs in commercial products is debatable, both amniotic membrane and amniotic fluid-derived products offer the opportunity to deliver growth factors and cytokines that can be used to treat a variety of orthopedic injuries. While the legal aspects regarding the use of such products are largely outside of the scope of this section, the concepts of minimal manipulation and homologous use as laid out by the FDA still apply. The processing and packaging methods of the amniotic membrane must be considered. Methods that compromise the original relevant characteristics of the tissue may change the product’s consideration as an HCT/P. Furthermore, while cells derived from secreted body fluids like amniotic fluid are considered HCT/Ps, the body fluids themselves are generally not considered HCT/Ps. Human clinical evidence on these commercial products for safety and efficacy for orthopedic issues is lacking as well.
Exosomes
Use of allogenic and autologous MSCs in interventional orthopedics has failed to completely fulfill clinical expectations due to concern over a lack of cell differentiation, loss of cells, and poor engraftment at target sites. , Research regarding the structure, makeup, physiologic, and pathologic function of the numerous substances secreted by MSCs (secretomes) has exponentially increased over the past several years. These unique MSC secretomes have anti-apoptotic and immunoregulatory properties and have been utilized for a wide variety of diagnostic and therapeutic applications.
Secretomes have both a vesicular and soluble part. The vesicular components secreted by MSCs are categorized as extracellular vesicles, and further subcategorized based on cellular origin, size, and biologic function. , A variety of extracellular vesicle subtypes have been described, including exosome, microvesicles, membrane particles, peptides, and cytokines. Exosomes are small, spherical particles (70 to 150 nm) that are enclosed by a phospholipid bilayer and capable of passing through a 0.1-μm filter. They were first described by Pan and Johnstone in 1983 and were initially believed to eliminate unwanted cellular components and waste during sheep reticulocyte maturation. , Recently, exosomes have received a great deal of interest due to their role in intracellular communication and immunomodulatory function, and their potential for use in identifying and treating a variety of disease states. ,
Exosomes originate from the cell membrane by endocytic internalization (budding inward). The most well-established mechanism of exosome biogenesis has three stages: the endosomes stage, the multivesicular bodies (MVBs) stage, and the exosomes stage. First, endocytic vessels are transferred to early endosomes—tube-like structures that are located near the outer edge of the cytoplasm. Early endosomes eventually mature into late endosomes, which are more spherical and located closer to the nucleus of the cell. Late endosomes, or MVBs, carry intraluminal vesicles that contain a variety of biomolecules, including proteins, lipids, and various types of RNAs. In the second stage, MVBs are either degraded by fusing with lysosomes or transported to the cell’s plasma membrane. Upon fusion with the plasma membrane, the MVB releases its contents into the extracellular space as exosomes—completing the third stage. In the extracellular space, recipient cells take up exosomes through paracrine or juxtracrine actions (exosomal fusion) or endocytosis ( Fig. 8.2 ).

The function and composition of exosomes are highly dependent on the physiologic state of the parent MSCs, where the MSCs are derived from, and the medium in which the MSCs are grown. , Exosomes act as cellular messengers conveying information to distant tissues through proteins and genetic material contained within that can ultimately alter the recipient cell’s physiology and function. Exosomes can be released by most cell types, normal and pathologic, and are present in conditioned cell medium in vitro, especially when derived from MSCs. They are stable in biologic fluids including blood, urine, saliva, breast milk, synovial fluid, and amniotic fluid. The majority of exosomes express surface protein Alix; Tsg 101; and transmembrane proteins CD9, CD63, and CD81, in addition to other proteins. ,
To be able to utilize exosomes for diagnostic or therapeutic applications, it will become necessary to establish efficient and effective methods to isolate exosomes with minimal alteration to their structure and/or content. Additionally, it is imperative to identify and only isolate exosomes with the desired trait. Several isolation methods have been developed and are currently in use. ,
The currently accepted gold standard for isolation of exosomes involves differential ultracentrifugation. This is performed by applying sequentially increasing centrifugal forces to a solution with exosomes to separate them from cells, large debris, and organelles. Ultracentrifugation is typically used in combination with sucrose density gradients or sucrose cushions to remove contaminants with densities different from exosomes. This method produces high-purity exosomal preparations, but is limited to only generating exosome-enriched samples. Pure exosome isolates are difficult to obtain due to the large number of similar-sized nanoparticles in samples.
As research continues to expand our knowledge regarding these cellular secretions, exosomes have the potential to become a truly disease-modifying orthobiologic drug with a number of clinical benefits. As a therapeutic tool, exosomes could potentially be produced and available as an “off-the-shelf” product with a defined composition, potency, and dose. These products could provide immediate bioavailability of the active allogenic agents, with consistent content and quality independent of the patient’s age and underlying health.
Based on basic science research, exosomes exhibit a range of biologic capabilities including regulation of angiogenesis and apoptosis, antigen presentation, and endocytosis. Through their miRNA contents, exosomes have the potential to regulate chondrocyte apoptosis and promote cartilage proliferation in the setting of osteoarthritis. In tendinopathies, the therapeutic application of exosomes has been shown to promote tendon healing by establishing a balanced microenvironment and increasing the expression of tenogenic markers. The ability of exosomes to play a crucial role in cell-to-cell signaling and mediate disease responses make them attractive options in regenerative medicine.
The legal considerations regarding these products remain out of the scope of this text; however, it should be noted that there are currently no FDA-approved exosome products. Given that these products require cell culture expansion, they are regulated as a drug as opposed to an HCT/P and are not approved for clinical use in the United States. Furthermore, to date there are no current human clinical trials evaluating the efficacy or safety of exosome treatments for any condition.
Of note, extracellular vesicles and exosomes have also been studied diagnostically. The presence of exosome-derived lncRNA has the potential to function as an indicator of osteoarthritis progression. Disease-state biomarkers are often low in bodily fluids and can be masked by other proteins in the sample. Extracellular vesicles can reflect the biologic state of the host cell, and if isolated have the potential to prove information of various disease states. While exosomes have immense potential diagnostically and are an ideal choice for biomarker analysis, this field is also still in its infancy make them.
Demineralized Bone Matrix
One of the most powerful tools in interventional orthopedics is in the percutaneous treatment of skeletal defects associated with fracture nonunion, osseous cysts, and avascular necrosis. In these conditions, the capacity for bone healing is diminished and, as a result, it is necessary to deliver bone grafts. Bone grafts are generally considered along two spectrums: osteoconductive and osteoinductive. Osteoconductivity refers to the ability of the material to act as a scaffold for the ingrowth of vessels and osteoprogenitor cells. , Osteoinductivity is the ability to promote the mitogenesis and differentiation of nearby cells into the osteogenic lineage. Bone grafts employing these factors must be combined with an appropriate source of progenitor cells that are either found in vivo in the surrounding perivascular tissue or delivered concurrently with the graft.
Autologous iliac crest bone graft is widely considered the gold standard in bone grafting of skeletal defects. It possesses all three characteristics of osteoconductivity, osteoinductivity, and osteogenic cells. Unfortunately, donor site morbidity and the high costs of graft harvesting can be limiting. Furthermore, iliac crest bone grafts are not easily delivered percutaneously. A valuable alternative to autogenous bone is the use of DBM ( Fig. 8.3 ). DBM is manufactured by acid digestion of bone, leading to a combination of collagens (predominantly type I), noncollagenous proteins, residual calcium phosphate, and residual cellular debris. DBM has a long history of clinical use dating back more than a century. The decalcification process used in the preparation of DBM causes the release of bone morphogenetic proteins into the surrounding tissue when it is implanted, leading to the osteoinductive properties of the material.
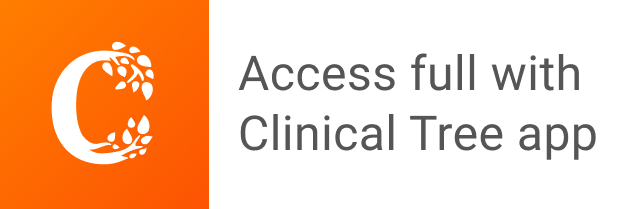