Pearls
- •
Safe management of the critically ill child’s airway requires a comprehensive understanding of the anatomic and physiologic changes that occur from birth through adolescence, advance recognition of congenital and acquired airway abnormalities, appreciation of the pathophysiologic consequences of airway manipulation, and preparation for airways that are potentially difficult to manage.
- •
Laryngoscopy and intubation are potent physiologic stimuli that are associated with severe discomfort, profound cardiovascular and cerebrovascular changes, and increased airway reactivity.
- •
Recognizing a difficult airway and preparing to manage it are essential to preventing potentially lethal complications of intubation.
- •
The approach to intubation must be tailored to specific circumstances, including full stomach, elevated intracranial pressure, cervical spine injury, and upper airway obstruction.
- •
Alternative approaches to airway management—such as the laryngeal mask airway, cricothyrotomy, retrograde intubation, tracheostomy, and video laryngoscopy—may be lifesaving.
The accurate assessment and safe management of the airway are the most essential first steps in providing effective care for a critically ill child. These steps require that the practitioner understand the anatomic and physiologic changes that occur from birth through adolescence, recognize congenital and acquired airway abnormalities, appreciate the pathophysiologic consequences of airway manipulation and underlying disease processes, and prepare for airways that may potentially be difficult to manage.
Anatomic considerations
Configuration of a child’s airway changes dramatically from birth to adulthood ( Fig. 127.1 ). The nose is the site of nearly half of the total respiratory resistance to airflow at all ages. The infant’s nose is short, soft, and flat, with small, nearly circular nares. The nasal valve, the narrowest portion of the nasal airway, is approximately 1 cm proximal to the alar rim in newborns and measures only about 20 mm 2 . , By 6 months, the dimensions of the nares have nearly doubled, but they are still easily occluded by edema, secretions, or external pressure. Although an infant is perhaps not as much the obligate nose breather as commonly assumed, signs of airway obstruction frequently develop when the infant’s nose is obstructed. ,

In infancy, the mandible is small and the basicranium (which provides the roof of the nasopharynx) is flat, creating a small oral cavity. Over the years of its development, the jaw grows primarily inferiorly and anteriorly, with the mandibular ramus increasing in height and width. The posterior portion of the basicranium develops a progressively more rounded configuration through childhood, which results in a larger nasal airway to meet the need for increased airflow. These anatomic changes provide a chamber for the resonance of adult speech.
Under normal conditions, the genioglossus muscle and other muscles of the pharynx and larynx help maintain airway patency. Both tonic and phasic inspiratory activity synchronized with phrenic contraction have been noted in animal and human studies. In particular, the genioglossus increases the dimensions of the pharyngeal airway by displacing the tongue anteriorly. In the infant and young child, the tongue is large relative to the surrounding bony structures and the cavities they form. Relatively little displacement is possible at any time. Therefore, loss of tone caused by sleep, sedation, or central or peripheral nervous system dysfunction is more likely to allow the tongue to relax into the posterior pharynx and cause upper airway obstruction in young children than it is in older patients.
The infant larynx is high in the neck at birth, with the epiglottis at the level of the first cervical vertebra and overlapping the soft palate. This approximation of structures, in combination with the relatively large tongue and small mandible, contributes to the vulnerability of infants and young children to airway obstruction. By 6 months, the epiglottis has moved to about the level of the third cervical vertebra and is separate from the palate. The epiglottis continues to descend to its adult position at about the fifth or sixth cervical vertebra by early adolescence. The infant epiglottis is soft and omega shaped, in contrast with the more rigid, flatter adult structure, and has greater potential to occlude the airway.
For many years, standard teaching has been that both the immature larynx and trachea differ greatly from the mature structures. However, modern imaging techniques, including bronchoscopy, computed tomography scanning, and magnetic resonance imaging, provide evidence that contradicts previous dogma. The immature larynx is roughly cylindrical, not funnel shaped, similar to the straight vertical column seen in adults. Although the narrowest part of the young child’s airway was previously thought to be at the level of the cricoid cartilage, studies show that, as in adults, it is at or just below the vocal cords. Nonetheless, the cricoid is more rigid than other areas of the airway and vulnerable to injury. At all ages, the trachea is slightly elliptical in cross-section, with the anteroposterior diameter slightly greater than the transverse diameter. These newer findings have important implications for endotracheal intubation and will be discussed later.
The internal dimensions of the trachea in a newborn are approximately one-third those of an adult, and absolute resistance to airflow is higher in newborns than in older children and adults. The most important factor determining resistance (R) is the radius (r) of an airway (Poiseuille’s law: R is proportional to 8 l/r ); therefore, small changes in airway diameter in infants or young children as a consequence of edema or secretions have a far greater effect on resistance than similar changes in larger patients (see Fig. 127.1 C).
Basic airway management
Optimal airway management is dependent on a brisk assessment of the patient’s breathing and prompt determination of the level of intervention required, if any. In virtually every setting in which respiratory difficulty is suspected, oxygen should be administered until the specific abnormality can be identified and adequately treated. Although extreme hypercarbia is usually well tolerated, hypoxia is routinely catastrophic and not always obvious on initial examination. The alveolar air equation clearly demonstrates that hypercarbia produces hypoxia at low fractions of inspired oxygen (Fi o 2 ; Table 127.1 ). If the patient is breathing spontaneously, attention should be directed first to signs of upper airway obstruction, including inspiratory and/or expiratory stridor, noisy breathing, lack of audible or palpable air flow, or a rocking chest and abdominal motion rather than the normal, smooth rise and fall that should occur with inspiration and expiration.
Alveolar gas equation | Pa o 2 = Fi o 2 (PB – PH 2 O) – Pa co 2 /0.8 |
Room air, normocarbia | Pa o 2 = 0.21 (760 – 47) – 40/0.8 = 99 mm H 2 O |
Room air, hypercarbia | Pa o 2 = 0.21 (760 – 47) – 80/0.8 = 50 mm H 2 O |
Supplemental O 2 , hypercarbia | Pa o 2 = 0.4 (760 – 47) – 80/0.8 = 185 mm H 2 O |
An alert child with normal neuromuscular function usually assumes a position that minimizes upper airway obstruction (tripod position) instinctively. However, a child with an altered level of consciousness or severe neuromuscular weakness may be unable to maintain a patent airway because of the inability to either alter the position or maintain adequate glossopharyngeal muscle tone.
Nasopharyngeal airway
A nasopharyngeal airway that extends through nasal passages to the posterior pharynx and beyond the base of the tongue is often adequate to relieve obstruction. It is tolerated by most patients, even those who are conscious ( Fig. 127.2 ). The largest airway that is able to pass through nasal passages without causing blanching of the skin surrounding the nares is extended from the nares to the tragus of the ear. The airway tube should be well lubricated before placement. Risks of nasopharyngeal airways include nasal ulceration, bleeding, laceration of friable lymphoid tissue, rupture of a pharyngeal abscess, laryngospasm, and potential passage through the cribriform plate in patients with basilar skull fractures. Topical vasoconstricting agents reduce but do not eliminate the risk of bleeding. Like other nasal tubes, use of nasal airways increases the risk of sinusitis; therefore, contraindications to their use include severe coagulopathy, cerebrospinal fluid (CSF) leaks, and basilar skull fractures.

Oropharyngeal airways
Oropharyngeal airways displace the base of the tongue from the posterior pharyngeal wall and break contact between the tongue and palate (see Fig. 127.2 ). Oropharyngeal airways can play an important role during bag-valve-mask ventilation if chest rise is absent or ineffective by relieving upper airway obstruction, which is the most likely culprit. Size selection is important. An excessively long airway may encroach on the larynx and cause laryngospasm. An airway that is too short may actually push the tongue posteriorly and exacerbate obstruction. In approximating ideal sizing, if the airway is held at the side of the face with the flange just anterior to the incisors, the tip should be at or near the angle of the mandible. The oropharyngeal airway should be positioned following the curve of the tongue while the tongue is held down and forward with a tongue depressor. Inserting the airway with its concave side facing the palate and then rotating it may traumatize the oral mucosa or damage teeth. Oral airways are poorly tolerated in any patient with a functional gag reflex and may induce vomiting. As a consequence, they are of little more than temporary value in the critically ill child. However, they are an important adjunct to help support a patent airway for bag-valve-mask ventilation in preparation for intubation.
Oxygen delivery devices
Nasal cannulas
Nasal cannulas consist of two hollow prongs projecting from a hollow face piece. Humidified oxygen (100%) flows from a standard source, effectively delivering a pharyngeal concentration of 25% to 40% after mixing with variable amounts of room air. The cannulas are easy to use, often readily tolerated, lightweight, economical, and disposable and take advantage of the humidifying properties of the nasopharynx. Flow typically is limited to only 3 to 5 L/min because of the extent to which relatively dry gas flow cools and dries the nasal airway. The use of nasal cannulas is limited by the relatively low oxygen concentration that can be delivered. Heated high-flow nasal cannulas (HHFNCs) can deliver up to 40 to 80 L/min of warmed, humidified gas. Flow is dependent on the size of the HHFNC, and this modality of oxygen delivery is usually well tolerated. The oxygen concentration delivered is higher than that with simple nasal cannulas. High-flow nasal cannulas generate positive distending pressure similar to that provided by nasal continuous positive airway pressure (CPAP). The pressure generated is dependent on the interaction among the flow rate, patient size, and anatomy of the patient’s airway, but it is probably limited to 4 to 5 cm H 2 O. , At least in infants, positive-pressure generation requires a closed mouth. Recently, the use of nasal cannula oxygen, particularly HHFNC as part of the preoxygenation plan to prepare critically ill patients for intubation, has been shown to prevent desaturation during the apneic phase of induction (this will be discussed at greater length later in this chapter).
Masks
A variety of masks are available for delivering oxygen. Simple masks fit loosely and the oxygen concentration delivered varies depending on the patient’s inspiratory flow rate and the oxygen flow into the system. Partial rebreathing masks incorporate some sort of reservoir, usually a bag below the chin. Provided that flow into the system exceeds the patient’s minute ventilation and the bag does not collapse on inspiration, little carbon dioxide (CO 2 ) is inhaled and concentrations of oxygen up to about 60% can be achieved. Nonrebreathing masks must fit snugly. They incorporate a mask, reservoir, and one-way valves that vent expired gas but do not permit inspiration of room air. As a result, they can deliver close to 100% oxygen.
Noninvasive positive-pressure ventilation
Noninvasive positive-pressure ventilation is defined as the delivery of mechanical ventilatory support without the use of an endotracheal or tracheostomy tube (invasive devices). It includes CPAP and bilevel positive airway pressure (BiPAP). CPAP delivers high concentrations of oxygen and maintains positive airway pressure in the spontaneously breathing patient. CPAP is applied with an oxygen source connected to either a tight-fitting nasal or full face mask or helmet in children or via nasal prongs in the neonate and older infant. CPAP offers the benefit of maintaining alveolar expansion and decreases work of breathing for many patients, particularly those with pulmonary parenchymal disease and some patients with airway obstruction related to poor upper airway tone or laryngeal-, tracheal-, or bronchomalacia.
Like CPAP, BiPAP can be provided by mask, but it requires a ventilator to assist with flow delivery. The patient’s inspiratory effort triggers the BiPAP machine to deliver decelerating flow in order to reach a preset pressure, defined as inspiratory positive airway pressure. When a patient’s own inspiratory flow falls below a preset amount, ventilatory assistance ceases and maintains expiratory airway pressure at a predetermined value (typically between 5 and 10 mm Hg). Uses in the pediatric intensive care unit (ICU) include upper airway obstruction, atelectasis, exacerbations of neuromuscular disorders, support for mild to moderate respiratory failure, and as an assist in weaning patients from invasive mechanical ventilation. Both CPAP and BiPAP offer the advantage of providing respiratory support without endotracheal intubation, but they require that the child tolerate a close-fitting mask. A more extensive discussion of CPAP and BiPAP is available in Chapter 55 .
Establishing a functional airway
A patient who is apneic or in severe respiratory distress requires ventilation assistance initially with a bag and mask. No skill is more essential for the intensivist than the ability to provide effective manual bag-mask ventilation. Bag-mask ventilation can be lifesaving while preparation for endotracheal intubation proceeds or when intubation cannot be accomplished. Effective bag-mask ventilation technique requires positioning the patient adequately to open the upper airway, achieving a tight mask-face seal, inserting an oral or nasal airway if needed, and generating an adequate tidal volume while coordinating manual breaths with patient efforts when they are present. Poor technique results in ineffective oxygenation and ventilation, gastric insufflation and distention, and increased risk of aspiration.
If the child is too weak or obtunded to maintain pharyngeal tone independently, the head should be placed on a thin cushion to cause slight cervical spine flexion and gentle extension at the atlanto-occipital joint. In infants, the large occipitofrontal diameter makes the cushion unnecessary and potentially disadvantageous, although a thin pad under the shoulders may be useful. It appears that aligning the external auditory meatus with the sternal notch is a reasonable guide to appropriate positioning. Current recommendations are to avoid overextending the baby’s flexible cervical spine, which may stretch and compress the trachea and potentiate, rather than relieve, obstruction. Studies have questioned the existence of this phenomenon but to date have included only a small number of infants, all with normal airways. Appropriate head tilt separates the tongue from the posterior pharyngeal wall. If airway obstruction persists, the chin can be pulled forward by encircling the mandible behind the lower incisors between the thumb and fingers. The most effective means of relieving functional obstruction is the so-called triple airway maneuver (head extension, jaw thrust, and mouth opening). With the fingers behind the vertical ramus of the jaw, the mandible is displaced downward, forward, and, finally, upward again until it and the lower incisors are anterior to the maxilla. This action effectively pulls the tongue forward and away from the pharyngeal wall.
In some patients, establishing a functional airway is sufficient to allow resumption of effective spontaneous ventilation. In other patients, steady positive airway pressure is necessary to overcome residual obstruction. If breathing remains inadequate, manual ventilation is necessary. Effective ventilation requires a good mask fit. The mask should sit smoothly on the bridge of the nose and the bony prominence of the chin. It is critical to avoid airway occlusion with the mask or hand or pressure on eyes, soft nasal structures, or branches of the trigeminal and facial nerves. An optimal mask seal is predictably difficult in a patient without teeth, a flat or prominent nose, or micrognathia. Insertion of a nasal or oropharyngeal airway may help maintain an adequate airway. Once a tight mask seal is ensured, ventilation may be assisted.
Two types of bags are in general use for bag-mask ventilation: self-inflating resuscitation bags and standard anesthesia bags. Self-inflating bags vary substantially; therefore, specific directions for their use must be followed carefully. All bags incorporate an adapter to connect to a mask or endotracheal tube (ETT), a bag, a pressure-relief valve, and a port for fresh gas inflow. Most bags made for children have pressure-relief valves designed to pop off at 35 to 45 cm H 2 O pressure to prevent excessive volume delivery and subsequent barotrauma. In patients with poor compliance or increased airway resistance, it may be necessary to bypass this valve temporarily to provide effective ventilation. Most systems incorporate valves that prevent rebreathing. Fresh gas flows through the valve on spontaneous inspiration (negative pressure) or on creation of positive pressure when the bag is squeezed ( Fig. 127.3 ). Exhaled gas is vented to the atmosphere. Not all systems allow spontaneous breathing. Those that do demand that the patient generate at least a little negative pressure; thus, a mask must fit well. Holding the mask above the patient’s face provides no supplemental oxygen. The percentage of oxygen delivered depends on the percentage of oxygen from the source, the fresh gas flow rate, and the respiratory rate, which determines the time available for the bag to refill. Most systems require some sort of reservoir assembly in addition to the self-inflating bag to prevent entrainment of room air. With a reservoir, 100% oxygen may be delivered; without a reservoir, most deliver less than 50%.

Anesthesia bags require flow from a source of gas under pressure in order to expand. Many variations have been reviewed extensively in the anesthesia literature. These circuits depend on the location of the fresh gas inflow and overflow valves, rate of fresh gas flow, the rate, tidal volume, CO 2 production, and whether ventilation is spontaneous or controlled. Many ICUs use the Mapleson D configuration, with the fresh gas source attached just distal to the patient connection. The overflow valve is proximal to the reservoir bag. During expiration, the patient’s exhaled tidal volume mixes with fresh gas flowing into the system and accumulates in the tubing and bag. With sufficiently high fresh gas flow, alveolar gas is washed to the overflow valve and eliminated from the circuit. To prevent rebreathing, the system requires higher fresh gas flow during spontaneous ventilation than during controlled breathing, but a safe rule of thumb is that fresh gas flow be two to three times the minute ventilation. During controlled ventilation, a minimum of 100 mL/kg per minute ensures that CO 2 elimination is proportional to minute ventilation. , At flows less than 90 mL/kg per minute, increasing ventilation may only increase CO 2 rebreathing.
Endotracheal intubation
The pediatric intensivist is frequently called on to intubate critically ill patients when brief periods of ventilation with a bag and mask are inadequate to address the underlying disorder. Few of these intubations are performed under the optimal conditions commonly attainable in the operating room—that is, relatively healthy children with empty stomachs who have previously been sedated and are intubated in a controlled environment with all members of the team experienced in and prepared for airway management. Instead, patients are often critically unstable and require intubation suddenly, commonly in settings where the procedure is not routine. Intubation often is viewed only as a means to an end, namely, mechanical ventilation. However, it is associated with profound physiologic consequences that may dramatically affect the patient. The intensivist’s appreciation of these factors and ability to minimize the adverse physiologic consequence of airway manipulation may determine patient outcome as decisively as the intensivist’s skill in providing the intensive care that follows.
Indications
Respiratory failure
Respiratory failure may result from dysfunction at any point along the ventilatory pathway. To provide appropriate support and to avoid hazards specific to the individual disorder, airway intervention must be tailored to the underlying cause. Outside the operating room, the need for intubation is most commonly associated with respiratory failure resulting from upper or lower airway or pulmonary parenchymal disorders that require mechanical ventilation. Respiratory failure is defined in terms of excessive work of breathing or inadequate oxygenation (in the absence of cyanotic congenital heart disease) or CO 2 elimination. Box 127.1 contains a set of criteria for intubation.
- •
Pa o 2 <60 mm Hg with fraction of inspired oxygen ≥0.6 (in absence of cyanotic congenital heart disease)
- •
Pa co 2 >50 mm Hg (acute and unresponsive to other intervention)
- •
Upper airway obstruction, actual or impending
- •
Neuromuscular weakness
- •
Maximum negative inspiratory pressure ≥20 cm H 2 O
- •
Vital capacity <12 to 15 mL/kg
- •
- •
Absent protective airway reflexes (cough, gag)
- •
Hemodynamic instability (cardiopulmonary resuscitation, shock)
- •
Controlled therapeutic (hyper)ventilation
- •
Intracranial hypertension
- •
Pulmonary hypertension
- •
Metabolic acidosis
- •
- •
Pulmonary toilet
- •
Emergency drug administration
Hemodynamic instability
Patients with hemodynamic instability often benefit from assisted ventilation. Positive-pressure ventilation decreases left ventricular transmural pressure and left ventricular wall stress, leading to afterload reduction. Controlled ventilation is often a critical component of cardiopulmonary resuscitation. Additionally, early intubation in anticipation of impending cardiovascular collapse may prevent catastrophic tissue hypoxia. Redistribution of blood flow away from respiratory muscles, especially the diaphragm, in patients with marginal cardiac output may improve perfusion of other vital organs, including the heart, and help prevent cardiac arrest.
Neuromuscular dysfunction
For additional information on neuromuscular dysfunction, see Chapter 46 .
Neuromuscular dysfunction or severe chest wall instability (or deformity) may cause failure of the bellows apparatus for breathing. Initially, tidal volume remains normal or at least sufficient to maintain normal blood gas tensions, but vital capacity and maximal inspiratory and expiratory pressures decrease. Inability to take a deep breath or cough forcefully risks progressive segmental or lobar atelectasis, inability to clear secretions, bronchial obstruction, and possible major airway obstruction with sudden severe hypoxia or CO 2 retention. Increasing weakness results in progressively smaller tidal volumes, loss of upper airway tone, and, ultimately, inadequate minute ventilation. Bulbar dysfunction may lead to aspiration as a result of impaired swallowing and inadequate cough.
Measurement of ventilatory reserve provides a better assessment of the patient’s need for ventilatory assistance than does arterial blood gas tension alone. Maximum negative inspiratory pressure and vital capacity are two simple tests commonly used for this purpose. A variety of other measures also help assess respiratory “strength,” but most are difficult to perform in sick, uncooperative infants and children. Patients with diffuse neuromuscular weakness of any cause, spinal cord dysfunction above the level of T6, or loss of phrenic nerve or diaphragm function are particularly prone to respiratory failure. Infants younger than approximately 6 months tolerate diaphragmatic paralysis poorly because of the high compliance of their chest walls and relative ineffectiveness of intercostal muscles.
Many patients with neuromuscular weakness respond well to noninvasive forms of ventilatory support. , Decisions about the best approach to airway management should be based on the nature and likely progression of the illness, the child’s maturity and level of consciousness, and the timing of the onset of respiratory insufficiency. In an emergency, endotracheal intubation is likely to be safest, with transition to noninvasive support when careful planning allows.
Failure of central nervous system to regulate ventilatory drive and airway reflexes
Failure of the central nervous system to regulate ventilatory drive may prompt intubation. Centrally mediated hypoventilation is manifested as CO 2 retention, usually in the absence of increased work of breathing. On occasion, the decision to support ventilation may be based on observing abnormal ventilatory patterns in anticipation of neurologic deterioration. Loss of protective airway reflexes, including the cough and gag reflexes, can result from central nervous system depression, cranial nerve abnormalities, or severe motor weakness. In such patients, intubation is indicated to prevent aspiration. Intubation may be appropriate when the practitioner anticipates a need to protect the airway and support ventilation during deep sedation for procedures or diagnostic studies.
Other indications
Intubation is indicated as a step toward therapeutic, controlled (hyper)ventilation (e.g., in patients with increased intracranial pressure [ICP] or pulmonary hypertension) or to support spontaneous hyperpnea in patients with metabolic acidosis and other conditions. Patients with profuse, thick, or tenacious secretions (e.g., as a result of bacterial pneumonitis or smoke inhalation) may benefit from having an artificial airway placed as a channel for effective suction. Mucociliary clearance is impaired in patients exposed to high oxygen concentrations or other airway irritants (including particulate and gaseous components of smoke), those experiencing severe hypoxia or hypercarbia, and, paradoxically, those who have airway trauma induced by endotracheal intubation and suctioning. Endotracheal intubation also provides an effective means of delivering drugs during cardiopulmonary resuscitation when venous or intraosseous access is not available. Finally, intubation may be warranted for nonemergent indications, such as bedside bronchoscopy or imaging studies, during which general anesthesia is needed to optimize image quality.
Physiologic effects of intubation
Laryngoscopy is a potent physiologic stimulus ( Box 127.2 ). , At the very least, it is a noxious stimulus that causes significant pain and severe anxiety, especially in children who cannot understand or accept the need for it. Laryngoscopy causes an increase in systemic blood pressure and heart rate initiated by pressure on the back of the tongue or lifting the epiglottis. , This effect is augmented by endotracheal intubation and suction. Nodal or ventricular dysrhythmias may occur. Sensory impulses that trigger this reflex probably are carried along the vagus nerve supplying the base of the tongue, epiglottis, and trachea. The efferent limb is less well defined but most likely the product of enhanced sympathetic activity. Infants respond more variably than do older patients. Hypertension develops in most patients, but a few become hypotensive, especially if they are hypoxic. Infants may demonstrate moderate to severe bradycardia rather than tachycardia, perhaps as a consequence of their greater parasympathetic tone. Sedation and light anesthesia decrease but do not eliminate the hypertension and tachycardia; topical anesthesia and deeper general anesthesia are more effective. Children with previous hypertension display an exaggerated vasopressor response. Sedation and neuromuscular blockade during airway manipulation in infants minimize the associated bradycardia and systemic hypertension. The impact of positive-pressure ventilation on cardiac performance depends on the underlying disorder (discussed in Chapter 32 ) and should be carefully considered in preparation for intubation.
Pain
Tachycardia
Anxiety
Bradycardia
Hypoxia
Systemic hypertension
Hypercarbia
Decreased systemic venous return
Increased intraocular pressure
Decreased jugular venous pressure return
Increased intragastric pressure
Increased intracranial pressure
Laryngospasm
Bronchoconstriction
Pulmonary hypertension
Laryngoscopy and intubation are potent stimulators of laryngospasm and may cause bronchoconstriction, especially in patients with a history of reactive airway disease. Increased airway resistance probably results from parasympathetic stimulation, with release of acetylcholine and stimulation of muscarinic receptors on airway smooth muscle, especially large central airways. Therefore, it is imperative to use adequate sedation and analgesia before laryngoscopy to prevent these complications.
During the intubation process, oxygen delivery to the patient is interrupted. Ineffective breathing or apnea increases the likelihood of hypoxia, especially in children, with their relatively low functional residual volume and higher basal metabolic rate. Patients with severe pulmonary disease and abnormally low functional reserve capacity are at particular risk. , During apnea, CO 2 tension increases at a rate of 3 to 4 mm Hg/min in healthy, sedated adults and probably more rapidly in children, particularly those with severe cardiopulmonary disease or increased metabolic rate resulting from fever, sepsis, or pain. , The process for optimizing oxygenation in preparation for laryngoscopy, also known as denitrogenation, is described in further detail later.
ICP rises immediately during laryngoscopy, even in patients without intracranial pathology, before changes in blood gas tensions occur. , , Cerebral metabolic rate and blood flow increase. Hypoxia, hypercarbia, and diminished jugular venous drainage, particularly in struggling patients, contribute further to increases in cerebral blood volume and increased ICP. Although normally transient, such intracranial hypertension may predispose patients with coagulopathies or vascular malformations to intracranial hemorrhage. Systemic hypertension in patients with impaired autoregulation of the cerebral circulation (e.g., sick infants or patients with a variety of intracranial disorders) and impedance to jugular venous return by jugular compression, pneumothorax, or coughing and struggling stress both the arterial and venous sides of the cerebral circulation. In patients with poor intracranial compliance, this effect is exaggerated and prolonged. In infants without primary central nervous system disease, muscle paralysis (even without sedation or analgesia) effectively blocks the rise in ICP associated with intubation. The systemic hypertensive response is generally unaffected by neuromuscular blocking agents (NMBAs) but can be modified by analgesia and sedation or intravenous anesthesia.
Patients with severe pulmonary hypertension are at high risk for adverse effects of laryngoscopy. Decreased oxygenation and progressive hypercarbia lead to elevated pulmonary artery pressure. The noxious stimulus of visualizing the airway in itself may precipitate life-threatening pulmonary hypertensive crisis.
Recognition of a difficult airway
Recognition of a difficult airway is important if potentially lethal surprises in airway management are to be minimized ( Box 127.3 ). Although the intensive care physician is usually focused on the immediate physiologic disturbances affecting the patient, careful preparation and an evaluation of each patient is critical. Key components of the history and physical examination, as well as the clinical scenario, can provide insight into potential problem airways. A history of difficult intubation in the past or episodes of upper airway obstruction (including snoring or sleep apnea) suggest structural abnormalities that may or may not be evident at the moment. Recent tonsillectomy and adenoidectomy, cleft palate repair, or any prolonged surgical procedure resulting in oral edema or bleeding increases the likelihood of difficulty. Examining facial structure is essential; inspecting the child’s profile is particularly important because significant abnormalities may not be fully apparent on frontal view alone ( Fig. 127.4 ). Certain genetic syndromes are associated with craniofacial anomalies, midline defects, or neuromuscular disorders that may make successful intubation via standard techniques exceptionally difficult. Treacher Collins syndrome (mandibulofacial dysostosis); Goldenhar syndrome (oculoauriculovertebral dysplasia); Down syndrome; Pierre Robin syndrome; and the mucopolysaccharidoses, such as Hurler syndrome and Hunter syndrome, are a few of the syndromes that produce characteristic features associated with a high probability of a challenging airway. Isolated micrognathia, macroglossia (glossoptosis), facial clefts, midface hypoplasia, prominent upper incisors or maxillary protrusion, facial asymmetry, a high arched narrow palate, small mouth, and short, muscular neck or morbid obesity are features that can interfere with effective bag-mask ventilation or visualization of the larynx. Limited temporomandibular joint or cervical spine mobility may make laryngoscopy and tube placement difficult. Midface instability or upper airway bleeding, edema, airway or neck masses, and foreign bodies are additional reasons for concern.
History
Difficult intubation
Upper airway obstruction, current or past, including snoring and sleep apnea
Anatomic features
Gross macrocephaly
Severe obesity
Facial asymmetry
Facial trauma
Midface hypoplasia
Airway bleeding
Small mouth
Oropharyngeal mass
Glossoptosis
Abnormal soft tissue infiltration
Midline clefts or high arched palate
Limited temporomandibular joint mobility
Micrognathia
Nasal obstruction
Limited neck mobility
Laryngotracheal abnormalities (congenital or acquired)

Several assessment systems assist with recognition and classification of the adult patient with a difficult airway. Although never validated in pediatrics, they provide a useful framework for assessing infants and children. The Mallampati classification ( Fig. 127.5 A) uses visibility of upper airway structures—particularly the uvula, soft palate, and faucial pillars—as a guide to the likely ease of intubation. Mallampati class 1 allows visualization of the uvula, soft palate, and faucial pillars. In class 2, faucial pillars and soft palate are visualized, but the base of the tongue obstructs the uvula. In class 3, only the soft palate is visualized. In class 4, the soft palate is not seen. Difficult intubation is more likely associated with classes 3 and 4. This scale can be used with cooperative children, and an approximate evaluation may be obtained by observing many crying infants and young children.

The Cormack laryngeal view grade score is shown in Fig. 127.5 B. The Cormack system requires an attempt to visualize the larynx; therefore, it is more valuable as a tool for describing difficulty once it has been encountered than for predicting it. The Cormack score is grade 1, full view of vocal cords and glottis; grade 2, partial view of vocal cords and glottis; grade 3, only the epiglottis is seen; and grade 4, the glottis and epiglottis are not visualized. Grades 3 and 4 predict difficult direct laryngoscopy. Although these classification systems are helpful in a controlled environment, particularly the preoperative area of a hospital, they are recognized as having limited utility in the emergent situations often encountered in the ICU, and their ability to predict the degree of difficulty with intubation in children is not well established.
Ability to visualize the faucial pillars, soft palate, and uvula usually predicts an uncomplicated intubation, but visibility may be difficult to assess in a sick, uncooperative child. Children with severe hypoxia, severe hypovolemia, or other causes of hemodynamic instability, such as intracranial hypertension, a full stomach, or a combination of these conditions, present added difficulties that must be considered.
When airway problems are anticipated, the intensivist should approach intubation with a plan specific to the difficulty noted and with a backup strategy in mind. The option of relocating a patient with anticipated difficult airway to the operating room should be discussed, depending on the patient’s stability and availability of operating room personnel at the time of intubation. Additional equipment should be on hand, including a variety of laryngoscope blades, forceps, ETT sizes, supraglottic airways, bronchoscopes, tracheostomy or cricothyrotomy trays, and additional skilled personnel as needed. Many institutions have developed difficult airway carts or packs and a team of highly skilled airway experts to assist when severe intubation challenges arise. , When choosing the approach to sedation, agents that can be reversed pharmacologically are preferred and should be titrated slowly to the desired effect. Fig. 127.6 shows a modification of the difficult airway algorithm of the American Society of Anesthesiologists, which provides an approach to managing the difficult airway. A similar plan is necessary at the time of extubation. Serious consideration should be given to extubating in the operating room or leaving an airway exchange catheter in place to facilitate reintubation if necessary.

Process of intubation
All equipment for intubation must be available before the procedure ( Fig. 127.7 ). Many critical care units have implemented intubation checklists intended to decrease peri-intubation injury or adverse effects. However, studies comparing the performance of a preprocedure checklist to usual care have yielded mixed results. , A source of suction and appropriate catheters, oxygen and necessary tubing, ventilation bag, mask, laryngoscope and proper-sized blade with a well-functioning light, ETTs of the expected size plus larger and smaller ones, airway forceps, stylet, and a means of securing the ETT should be present at the head of the bed so that the intubator never needs to look away from the patient. A functioning intravenous catheter for drug infusion is essential in all but the most extreme emergencies.

Prior to laryngoscopy, a decision should be made regarding use of video laryngoscopy or standard laryngoscopy. Video laryngoscopy, as detailed later in this chapter, provides multiple benefits but must be weighed against immediacy of availability and potential disadvantages. Laryngoscope handles are available in standard adult and pediatric sizes. The smaller diameter of the pediatric handle makes it easier to manipulate, particularly when intubating infants and very young children. Blades of many types are available. The most important characteristic is length. Inexperienced operators often select a blade that is too short, making visualization of the larynx difficult. Excessively long blades make it difficult to avoid pressure on the upper lip and teeth. Historically, straight blades have been recommended for intubating infants and young children, but recent evidence suggests that curved blades (e.g., Macintosh no. 1 or 2 blades) are as likely to provide good visualization. The slightly curved tip of the Miller blade makes visualization of the larynx possible without actually lifting the epiglottis. The broader blade and bore of the Wis-Hipple blade help displace soft tissues in the young infant’s oropharynx. The Miller no. 2 blade is especially versatile in a broad age group (children ages 3 to 10 years). In older children, use of a curved blade often works best. If a cuffed ETT is to be used, a curved Macintosh no. 2 or 3 blade is effective in most patients and may provide more room to manipulate a cuff in the oropharynx. Operator experience is probably the most important factor that determines intubation success as well as willingness to try an alternative blade if one provides a poor view of the airway.
Selecting the proper tube size (diameter) is important for achieving effective mechanical ventilation and preventing tracheal injury. A variety of formulas are in use, with the most common being the Cole formula: Tube size (inner diameter) = (Age [years]/4) + 4. Cuffed tubes typically should be a half size smaller. For infants, no formula is accurate. Table 127.2 gives reasonable guidelines. Individual differences require that the tube size be modified for each child so that the tube passes easily but fits snugly enough to allow delivery of adequate mechanical breaths at a given chest compliance.
Age | Internal Diameter | Orotracheal Length (cm) | Nasotracheal Length (cm) |
---|---|---|---|
Premature | 2–3 | 6–8 | 7–9 |
Newborn | 3–3.5 | 9–10 | 10–11 |
3–9 mo | 3.5–4 | 11–12 | 11–13 |
9–18 mo | 4–4.5 | 12–13 | 14–15 |
1.5–3 y | 4.5–5 | 12–4 | 16–17 |
4–5 y | 5–5.5 | 14–16 | 18–19 |
6–7 y | 5.5–6 | 16–18 | 19–20 |
8–10 y | 6–6.5 a | 17–19 | 21–23 |
11–13 y | 6–7 a | 18–21 | 22–25 |
14–16 y | 7–7.5 a | 20–22 | 24–25 |
Based on traditional teaching about airway anatomy, cuffed ETTs were viewed as unnecessary or inappropriate in young pediatric patients (<8 years). However, better recognition of the elliptical shape of the young child’s airway shows that a tube that permits a small leak around itself (as previously recommended) may still compress the lateral aspects of the tracheal mucosa (see Fig. 127.1 D). Cuffed tubes allow better occlusion of the airway and potentially place less pressure on the mucosa while offering better oxygenation and ventilation, more reliable end-tidal CO 2 monitoring, and less risk of aspiration. Routine use of cuffed tubes is increasing for patients of all ages as a greater variety enters the market. In addition, the greater elastic recoil of the lungs and chest wall of older patients may demand higher airway pressures for effective ventilation.
Cuffed tubes of all sizes are available and are especially useful in patients who require consistent minute ventilation (e.g., those with severely elevated ICP or reactive pulmonary vasculature) or relatively high airway pressures. Increasing evidence indicates that cuffed tubes can be used in young children without higher incidence of airway complications. It appears that ideal tubes have clear depth markers along their length and an ultrathin, polyurethane, high-volume, low-pressure, spherical rather than elliptical cuff, positioned close enough to the tip of the tube to avoid inflation in the larynx. When a cuffed tube is used, great care should be taken to inflate it with the minimum occlusive volume , the minimum volume required to just seal the gas leak around the tube during mechanical inspiration and prevent mucosal ischemia and subsequent tracheal damage. Our current best understanding is that pressure within the cuff should be maintained below 20 to 30 cm H 2 O. There are inexpensive devices for measuring cuff pressure built into syringes used to fill the cuff. In addition, a standard pressure transducer with air-filled tubing can be attached to the pilot balloon on the ETT and attached to a typical ICU monitor for continuous pressure measurements. Cuffed tubes may be advantageous because they decrease the likelihood of needing multiple intubations to identify the correct size and do not need to be changed in a critically unstable patient if lung disease worsens. In addition, absence of a significant leak around the ETT may decrease the likelihood of flow-triggered ventilator autocycling. The ability to occlude the leak also facilitates pulmonary function testing and indirect calorimetry.
Pharmacologic agents to facilitate intubation
With the exception of intubation during cardiopulmonary resuscitation, the physiologic and psychologic benefits of using analgosedation before intubation necessitate administration during almost all clinical scenarios. , An analysis of data in the pediatric National Emergency Airway Registry shows that the intubation success rate is higher when both sedation and neuromuscular blockade are used. This finding is equally true in neonates, in whom sedation and neuromuscular blockade are still not used consistently. Most evidence in neonates indicates that use of neuromuscular blockade is associated with a lower risk of intracranial hemorrhage and pulmonary airleak. Excellent technical airway skills are an absolute prerequisite, however, because losing control of the airway invites catastrophe. Drugs that facilitate intubation are listed in Table 127.3 .
Drug | Dose | Duration | Comments |
---|---|---|---|
Intravenous Anesthetics | |||
Etomidate | 0.3 mg/kg IV | 3–5 min | Anesthesia, adrenal suppression (↑ mortality in sepsis?), minimal CV effect, apnea, ↓ CMR O 2 , ↓ CBF, ↓ ICP |
Ketamine a | 1–2 mg/kg IV; 4–6 mg/kg IM | 10–15 min | Anesthesia, ↑ systemic arterial pressure, ↑ HR, ↑ ICP, ↑ IOP, hallucinations, laryngospasm, bronchodilation |
Propofol | 1–3.5 mg/kg IV, then 0.05–0.3 mg/kg/min | 10–15 min | ↓ Systemic arterial pressure, ↓ CMR O 2 , ↓ CBF, ↓ ICP, metabolic acidosis |
Sedatives/Analgesics | |||
Fentanyl a | 2–5 μ/kg IV | 30–90 min | Analgesia, respiratory depression, CV stability, occasional bradycardia, or chest wall rigidity |
Remifentanil | 1–3 μg/kg, then 0.25–1 μg/kg/min | Analgesia, respiratory depression, CV stability | |
Morphine a | 0.1–0.2 mg/kg IV | 2–4 h | Analgesia, respiratory depression, ↓ systemic arterial and venous tone, ↓ systemic blood pressure |
Midazolam a | 0.1–0.3 mg/kg IV | 1–2 h | Amnesia, sedation or euphoria, ± CV stability, occasional respiratory depression |
Lorazepam | 0.1–0.3 mg/kg IV | 2–4 h | Sedation, anxiolysis, minimal CV effect |
Neuromuscular Blocking Agents | |||
Rocuronium a | 0.6–1.2 mg/kg IV | 15–45 min | Minimal cardiovascular effect, prolonged duration in liver failure |
Vecuronium a | 0.1–0.3 mg/kg IV | 30–75 min | Minimal cardiovascular effect, prolonged effect in hepatic failure |
Cis-atracurium | 0.1 mg/kg, then 1–5 mg/kg/min | 20–35 min | Metabolized by plasma hydrolysis, mild histamine release |
Atracurium | 0.5 mg/kg | 30–40 min | Metabolized by plasma hydrolysis, mild histamine release |
Succinylcholine a | 1–4 mg/kg IV | 5–10 min | ↓ HR, K + release in neuromuscular disease, trauma or burns, masseter spasm, malignant hyperthermia, myoglobinuria |
a Agents may be given intramuscularly but will have slower onset and more variable duration of effect.
Anticholinergic agents
Anticholinergic agents decrease oral secretions and prevent bradycardia, particularly in young infants, although their use is not universally recommended. , Atropine (0.02 mg/kg) and glycopyrrolate (0.01 mg/kg intravenously) are both immediately effective. Glycopyrrolate does not cross the blood-brain barrier and may be useful in patients who have suspected traumatic brain injury with bradycardia and require monitoring by pupillary examination. Scopolamine provides amnesia, decreases secretions, and prevents bradycardia but has a longer duration of onset. The drying effect of all anticholinergics commonly requires approximately 15 to 30 minutes and is rarely achieved in emergency intubation. Pediatric advanced life support guidelines (2011) recommend atropine for the following indications: (1) rapid-sequence intubation for infants under 1 year of age, (2) patients 1 to 5 years of age receiving succinylcholine, (3) patients older than 5 years receiving a second dose of succinylcholine, and (4) patients with bradycardia present before intubation. These guidelines do not provide information regarding patients older than 1 year who are not receiving succinylcholine. Therefore, clinical judgment on a case-by-case basis is essential for determining when to use anticholinergics. For example, a 3-year-old child with a heart rate of 60 beats/min may benefit from receiving atropine before intubation. In contrast, a 10-month-old with a heart rate of 240 beats/min may not require administration prophylactically; instead, atropine should be readily available as needed.
Sedative and analgesic agents
Most patients benefit from some degree of sedation for both comfort and facilitation of ideal intubating conditions. Drugs commonly used include intravenous anesthetic agents, anxiolytic agents, and opioid analgesics. The appropriate choice in a particular patient depends on the child’s hemodynamic status, level of anxiety, and underlying disease process. One of three intravenous agents is usually appropriate to facilitate amnesia and ideal intubating conditions: etomidate, propofol, or ketamine.
Etomidate is a short-acting intravenous anesthetic that causes rapid loss of consciousness (at 0.3 mg/kg) and respiratory depression. It also decreases cerebral oxygen consumption, cerebral blood flow (CBF), and ICP, without causing significant detrimental effects on cardiovascular function. It causes less respiratory depression than thiopental—an agent widely used in the past but no longer readily available in the United States. These characteristics have led to the common use of etomidate for emergency intubation. , It is important to note that etomidate has no analgesic properties and is not titratable; however, intubation doses of etomidate are amnestic and induce a state of general anesthesia. Adverse effects include vomiting, myoclonus, and lowering of the seizure threshold. Etomidate can cause adrenal insufficiency, making it inappropriate for long-term use in the ICU. Growing evidence suggests that it may suppress adrenal function even after a single dose, particularly in patients with sepsis and shock, raising questions about its use in these settings. However, the favorable hemodynamic profile of etomidate may outweigh the risk of adrenal insufficiency, which can be followed and treated with corticosteroids as needed. The hemodynamic stability is particularly relevant in cases of septic shock or catecholamine depletion.
Ketamine is another potent nonnarcotic analgesic and anesthetic that has been used safely in children in the critical care setting. , It is an especially useful sedative agent when maintenance of spontaneous ventilation is desired, as ketamine is associated with a low risk of apnea when used in isolation. Ketamine increases heart rate, systemic blood pressure, and cardiac output and is a fairly potent bronchodilator. However, myocardial depression may be apparent after administration to patients with catecholamine depletion. In a multicenter, prospective observational cohort study, ketamine use in patients with unstable hemodynamics was not associated with a lower prevalence of new hypotension. Ketamine may be of particular value in patients with status asthmaticus or other reasons for bronchospasm and may have a beneficial effect in sepsis. Although spontaneous ventilation is preserved in most patients, laryngospasm may occur. Given the risk of laryngospasm, it is important to note that ketamine is a sialogogue. Anticholinergic agents, such as glycopyrrolate and atropine, can be used to prevent/reduce the hypersalivation caused by ketamine. In the past, ketamine was considered inappropriate for patients with intracranial hypertension because of evidence that it increases cerebral metabolic rate, blood flow, and ICP. More recent studies indicate that it may be used safely in these patients, but no clear consensus has emerged. Emergence delirium and hallucinations occur frequently and may be prolonged and recurrent, particularly in adolescents and young adults. Ketamine has been used successfully for a variety of procedures in children, with few reported neuropsychiatric complications, but follow-up in most studies has been short and superficial. Whereas most patients do not suffer severe disturbances, those who do may have severe and prolonged distress. Benzodiazepines or barbiturates may decrease the incidence and severity of such adverse effects and the incidence of vomiting, although the data in children are limited and conflicting. , , , ,
Propofol is an ultra–short-acting agent with rapid onset and offset unless given by continuous infusion. It causes respiratory depression, desaturation, and systemic hypotension secondary to its negative inotropic effects and peripheral venous and arterial vasodilation. Its role in airway management of critically ill children is limited because of these effects. It has gained widespread acceptance as an anesthetic agent in children, however, and has been used extensively for procedural sedation. , Use in the ICU for more than approximately 6 hours is not recommended because of its association with metabolic acidosis, cardiovascular collapse and death, and propofol infusion syndrome among pediatric ICU patients. Current labeling warns against its use for prolonged sedation in children.
The benzodiazepines—including diazepam and midazolam—relieve anxiety, produce sedation in most children, and provide amnesia for noxious procedures. They do not relieve pain. Benzodiazepines have relatively little hemodynamic effect in most patients (except for those with myocardial depression) and rarely interfere with spontaneous breathing at therapeutic doses when used in isolation. Benzodiazepines decrease cerebral oxygen consumption modestly and, when used for intubation, are best combined with an opioid analgesic to decrease the discomfort and pain associated with laryngoscopy and passage of the tube.
Opioids commonly used for intensive care include morphine, fentanyl, and some of the ultra–short-acting agents, such as remifentanil. They cause respiratory depression in a dose-dependent fashion and increase intracranial blood flow in proportion to the increase in partial pressure of arterial carbon dioxide (Pa co 2 ). If hypercarbia is prevented, opioids decrease cerebral metabolic rate and blood flow. In the setting of altered cerebral autoregulation, they may not protect the patient from alterations of CBF. , Morphine may lead to histamine release with peripheral vasodilation and may precipitate systemic hypotension. Fentanyl is approximately 100 times more potent than morphine but does not cause histamine release and has little hemodynamic effect, even at anesthetic doses. However, large doses given rapidly can cause bradycardia or chest wall rigidity; therefore, the ready availability of a short-acting NMBA is critical. Remifentanil is a rapid-onset, ultra-short-acting opiate that is even more potent than fentanyl and may have potential benefit during intubation for procedures. In some patients with severe hemodynamic instability, a titrated opioid-only approach may be appropriate.
Neuromuscular blocking agents
Whether to use neuromuscular blockade and which NMBA to use are important considerations for the pediatric intensivist preparing to intubate a critically ill child. NMBAs cause reversible paralysis that facilitates visualization of the airway and insertion of the ETT in an atraumatic fashion. NMBAs are divided into two major categories: depolarizing and nondepolarizing agents. Nondepolarizing NMBAs are the most commonly used by pediatric intensivists. Differences between specific agents are primarily in their hemodynamic effects, metabolism, and excretion. , Among nondepolarizing NMBAs, the aminosteroid agent rocuronium is most commonly used for facilitation of intubation in critically ill patients. Rocuronium rapidly provides ideal intubating conditions nearly as rapidly as succinylcholine (in ∼45–90 s) without the adverse effects. Its duration is longer, lasting 15 to 45 minutes (and longer in infants). Rocuronium has minimal hemodynamic effect, is metabolized by the liver, and is largely excreted in bile (with a small amount excreted by the kidneys). Similar to rocuronium, vecuronium has virtually no hemodynamic effect. Its duration of action varies depending on the patient’s age, approximately 70 minutes in infants and 35 minutes in older children. Vecuronium is metabolized exclusively by the liver. Atracurium and cis-atracurium, both benzylquinolinium agents, also have minimal hemodynamic effects in most patients but may cause histamine release and hypotension in some. Metabolism occurs by spontaneous plasma hydrolysis; thus, neither renal nor hepatic function is necessary for elimination. Duration of action is short at about 15 to 20 minutes.
The only depolarizing NMBA in clinical use is succinylcholine. Its only advantage is its rapid onset of action (45–60 s) and brief duration of action (5–10 min). Muscle fasciculations occur at the onset of action in patients older than 4 years and may increase intracranial, intraocular, and intragastric pressure. Defasciculating doses of a nondepolarizing neuromuscular blocker prior to succinylcholine administration minimize such effects. Massive hyperkalemia may occur after its use in patients with spinal cord injury, severe burns, crush injuries, or neuromuscular disease. More recently, the spread of acetylcholine receptors outside of the neuromuscular junction, the mechanism presumed to underlie the massive hyperkalemic response, has been recognized to occur in many forms of critical illness associated with immobility, placing many critically ill patients at risk. Succinylcholine is a known trigger for malignant hyperthermia, even in the absence of volatile anesthetic exposure, and is a particular risk to patients with previously undiagnosed myopathies, often associated with abnormality in the ryanodine receptor type 1 gene ( RYR1 ). It frequently causes myoglobinuria in otherwise healthy children. The US Food and Drug Administration has issued a warning against succinylcholine use for routine intubation in children because of these complications. Although it is frequently used for emergency intubations and is widely recommended, , , the difference in time to conditions for intubation between succinylcholine and rocuronium is small (∼30 s), rarely of clinical significance, and inadequate to justify the added risk in most cases. The time to critical hemoglobin desaturation in the case of a failed airway is shorter than its duration of action, especially in children. Therefore, its shorter duration of action does not provide a meaningful advantage over nondepolarizing blockers.
In the past, scenarios that required rapid onset of neuromuscular blockade but short duration (e.g., the unanticipated difficult or critical airway) often called exclusively for succinylcholine. However, sugammadex, a unique neuromuscular blockade reversal agent for aminosteroid nondepolarizing blockers, is now available for immediate reversal of deep neuromuscular blockade. Sugammadex, unlike neostigmine, does not inhibit acetylcholinesterase; thus, it does not have to be coadministered with antimuscarinic agents (glycopyrrolate or atropine). Sugammadex works by binding up the relaxant agent; it has a greater affinity for rocuronium than for vecuronium, though it can be used to reverse both. In published studies, sugammadex was 10.22 minutes (6.6 times) faster than neostigmine in reversing moderate induced paralysis and 45.78 minutes (16.8 times) faster than neostigmine in reversing deep induced paralysis. A more extensive discussion of anesthetic agents and their use is given in Chapters 130 and 131 .
Preoxygenation
Spontaneous or manual ventilation with supplemental oxygen is maintained as drugs to facilitate intubation are given. Many patients who require emergency intubation have severely impaired gas exchange and may need to breathe 100% oxygen for several minutes, often with positive inspiratory and end-expiratory pressure. An integrative literature review identified 14 studies that showed benefits of passive apneic oxygenation during emergency intubations. During the apneic phase of emergency intubations, passive oxygenation via nasal cannula oxygen (standard or heated high flow) may prevent hypoxia or improve oxygen saturation, allowing for safe extension of apnea time and improved first-pass attempt success rate. Three minutes of spontaneous breathing at 100% FiO 2 allows denitrogenation with alveolar oxygen fraction close to 95% in patients with normal lung function. Tolerable apnea time, defined as the delay until the peripheral capillary oxygen saturation reaches 90%, can be extended up to almost 10 minutes after 3 minutes of classic preoxygenation. Some ICUs are starting to use noninvasive ventilation (BiPAP and continuous positive airway pressure [CPAP]) for preoxygenation before intubation. It is important to note, however, that a large multicenter randomized controlled trial of 2000+ adult patients in France did not show a reduction in the risk of severe hypoxemia in patients with acute hypoxemic respiratory failure when preoxygenation was used with noninvasive ventilation during intubation.
Orotracheal intubation
When all equipment is ready, an assistant is assigned to monitor the child’s color, heart rate, blood pressure, and oxygen saturation and to administer drugs when ordered. The child is placed supine with the head in the “sniffing” position. The infant’s large occipitofrontal diameter naturally results in good position most of the time, but a small pad under the shoulders may be helpful. In older children, a thin pad under the occiput helps establish slight neck flexion ( Fig. 127.8 ). The head is extended to align the oral, pharyngeal, and laryngeal axes as much as possible. Applying cricoid pressure during manual ventilation helps to minimize gastric distention by air ( Fig. 127.9 ). After the medications take effect, the pharynx is suctioned and stomach contents are aspirated. The patient is again briefly oxygenated, and the mask is removed. In a fully relaxed patient in good position, the mouth falls open. It can be opened more widely with caudad pressure on the chin by the intubator’s left fifth finger as the laryngoscope is introduced into the right corner of the mouth. If the patient is not sedated or the mouth opens abnormally, it may be necessary to open the jaw with the often-recommended scissor-like use of the right thumb and forefinger.
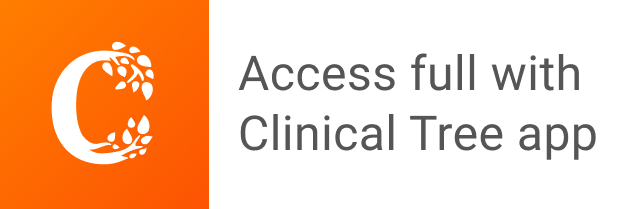